Impacts of ocean warming on a reef-building coralline alga Amphiroa cf. fragilissima under high irradiance
- 1Key Laboratory of Tropical Marine Bio-resources and Ecology, South China Sea Institute of Oceanology, Chinese Academy of Sciences, Guangzhou, China
- 2Southern Marine Science and Engineering Guangdong Laboratory, Guangzhou, China
Coralline algae, an important calcifying group, play vital roles in the primary productivity, reef frameworks construction, and carbon store. In this study, we investigated the responses of an articulated coralline alga Amphiroa cf. fragilissima to ocean warming under various light intensities. The relative growth rate significantly decreased under light or heat stress. When A. cf. fragilissima was exposed to high light intensity (120 μmol photons m−2 s−1) at 32°C, the relative growth rate was lowest, which reduced by 87% compared with that of group A1 (60 μmol photons m−2 s−1, 26°C). Meanwhile, a higher level of algal bleaching occurred when light intensity was 120 μmol photons m−2 s−1. Similarly, Fv/Fm and Chl-a content were negatively affected by light and heat stress, but they were more affected by light. Furthermore, the mineralogical responses to temperature and light were investigated. The net calcification rate declined from 92.27 (60 μmol photons m−2 s−1, 26°C) to 10.92 μmol CaCO3 g−1 DW day−1 (120 μmol photons m−2 s−1, 32°C). High temperature significantly decreased Ca content in live algal pigmented layer, whereas there were no significant differences in Ca content in the skeleton layer, implying that the pigmented layer could protect skeleton layer from mineral changes under ocean warming. The results revealed that A. cf. fragilissima was impaired by high light or thermal stress from various aspects, including growth, survival, photosynthesis, reproduction, and calcification. This study contributes to understand the effects of warming and light on coralline algae and provides a theoretical basis to protect the richness and diversity of calcified macroalgae.
Introduction
Annual global temperature has warmed 1.2°C between 1880 and 2020 (NASA/GISS). It is predicted that the global average air temperature will be warmer with an increase of 1.8°C–5.7°C by 2100 (IPCC, 2021). Such high increase in the temperature may bring serious negative threats on several marine calcifiers (Martone et al., 2010; Vásquez-Elizondo and Enríquez, 2016; Sully et al., 2019). Meanwhile, there are pieces of evidence that light availability is another important environmental factor affecting metabolic processes, such as growth, calcification, and photosynthesis of marine calcifiers (Suggett et al., 2013; Williams et al., 2018; Levy et al., 2020). The light intensity varied with depth, weather conditions, and turbidity. Generally, high irradiances and increased temperature occur together. Furthermore, there is a synergistic influence of light stress and heat stress on marine calcifiers (Downs et al., 2013; Rosic et al., 2020). Rosic et al. (2020) have reported that thermal stress under low light intensity induces higher levels of bleaching in coral Acropora millepora, which may eventually affect the diversity, abundance, and ecological functions of marine ecosystems. Therefore, there is an urgent need to investigate the responses of marine calcifiers to elevated temperature under various light intensities.
Coralline algae, a predominant calcifying group, are widespread in marine ecosystems from the intertidal zone to the deepest depths recorded for any attached autotroph (Steneck, 1986; Dean et al., 2015). They play important roles in the reef frameworks construction, carbon store, and sulfur cycle (Burdett et al., 2015; Van der Heijden and Kamenos, 2015; Jørgensbye and Halfar, 2017). Particular genera or species of coralline algae even form the massive algal ridges or rim on the high energy windward side. For example, the rhodolith beds were observed in Greeland, which could protect the inner reef and shoreline from wave energy (Jørgensbye and Halfar, 2017). Furthermore, certain species can induce the settlement of coral larvae and keep their surfaces free of opportunistic seaweeds and other epiphytes (Gomez-Lemos and Diaz-Pulido, 2017; Yang et al., 2020). Previous studies have revealed that the distribution, diversity, and ecosystem functions of coralline algae are affected by light availability, temperature, depth, and water motion (McCoy and Kamenos, 2015; Sañé et al., 2016; Sarkar, 2017). However, the study in which light variability modulates the physiological effects of warming on coralline algae has been limited (Comeau et al., 2013; Vásquez-Elizondo and Enríquez, 2016).
There have been 37 articles that investigate the physiological effects of ocean warming on coralline algae (Yang et al., 2021a). The studies have revealed that the algal physiological responses, especially calcification and photosynthesis, to warming are highly variable, including negative (Vásquez-Elizondo and Enríquez, 2016) or positive (Campbell et al., 2016), as well as no significant response (Tanaka et al., 2017; Cornwall et al., 2019). The different responses to increased temperature may be related to species-specific physiologies, geographic regions, treatment time, and algal life-history stages. Rendina et al. (2019) have revealed that calcification of Corallina officinalis is enhanced at 5 weeks but decreases at 7 weeks at high temperature, implying that a prolonged exposure to high temperature negatively affects the algal physiology. It is reported that the sensitivity of sporelings of Porolithon onkodes to warming is higher than that of adult thalli (Ordoñez et al., 2017; Tanaka et al., 2017). Unfortunately, among these studies, the comprehensive effects of irradiance availability and warming on coralline algae have rarely investigated (Comeau et al., 2013; Vásquez-Elizondo and Enríquez, 2016). Vásquez-Elizondo and Enríquez (2016) have reported that the light-induced photodamage is exacerbated under elevated temperature for Neogoniolithon sp., Amphiroa tribulus, and Lithothamnion sp. Furthermore, to our knowledge, there is currently very little information available on the mineralogical responses of coralline algae to warming under different light intensities.
The genus Amphiroa is important coralline algae in many shallow, near-shore exposed and tide-pool environments (Wai, 2018), which may experience different light intensities due to their distribution depth. They can fill the crevices in the reef structure and provide sand to the shore line via their calcified structures (Littler and Littler, 2013). Moreover, several species induce larval metamorphoses of Haliotis asinina (Williams et al., 2008). In our previous study, the impacts of temperatures on the growth and calcification rates of A. cf. fragilissima were investigated within 16 days. The results revealed that these physiological processes were significantly inhibited when the temperature was more than 32°C (Yang et al., 2021b). However, it is unknown about the photosynthesis and mineralogical responses when A. cf. fragilissima has prolonged exposure to warming under different light intensities. Here, we address two key questions. First, we investigated the physiological (growth, photosynthesis, chlorophyll, and calcification) and microstructure responses of A. cf. fragilissima to three different light intensities during heat stress. Second, we assessed and predicted the mineralogical changes at microscale under a combination of high temperature and light intensity treatments using scanning electron microscopy–energy-dispersive spectrometer (SEM-EDS) and X-ray diffraction (XRD).
Material and methods
Samples collecting and experimental design
Amphiroa cf. fragilissima was collected from coast of Sanya City (18°13′ N, 109°2′ E), Hainan Island in South China in December according to the method of Yang et al. (2021b). The temperature was approximately 26°C in December, whereas the mean monthly temperature was 22°C–30°C at this location (Jiang et al., 2017). The clean algal samples were placed into the filtered natural seawater collected from the sampling site to acclimate the experimental control conditions (60 μmol photons m−2 s−1, 26°C, pH 8.05 ± 0.01, 35 psu, 12:12-h cycle from 8:00 to 20:00). The values of Fv/Fm were 0.58 ± 0.02, 0.55 ± 0.02, and 0.57 ± 001 at days 1, 4, and 7, respectively. After day 7, thalli were applied to the experimental manipulation.
The treatments included two temperature levels (ambient control, 26°C; and elevated, 32°C) and three light intensities (60, 80, and 120 μmol photons m−2 s−1). In December, 26°C was the ambient temperature, whereas 32°C was 2°C higher than the mean monthly maximum temperature at the sampling site. There were six treatments in total, including A1 (26°C, 60 μmol photons m−2 s−1), A2 (26°C, 80 μmol photons m−2 s−1), A3 (26°C, 120 μmol photons m−2 s−1), A4 (32°C, 60 μmol photons m−2 s−1), A5 (32°C, 80 μmol photons m−2 s−1), and A6 (32°C, 120 μmol photons m−2 s−1). The elevated temperature was reached using one water heater (Aqua One, Glass Aquarium Heater, 100 W) per aquarium. There were three replicate tanks (12-L tank with 9 L of seawater) per treatment, with each tank containing approximately 20–25 g of wet thallus. There were 18 experimental tanks in total. All tanks and thallus were randomly allocated to treatments. During the experiment, all thallus were exposed to same conditions (pH, 8.05 ± 0.01; salinity, 35 psu) with 12:12-h (light:dark) cycle from 8:00 to 20:00 except temperature and irradiance. The filtered natural seawater was gently aerated with submerged pumps at 5 L min−1 in each tank and changed twice per week. The experiment ran for 4 weeks. The seawater salinity, pH value, and temperature of each tank were monitored daily with a calibrated handheld YSI meter (YSI, Yellow Springs, OH, USA). The values of salinity, pH, and temperature were listed in Figure S1.
The relative growth rate and photosynthetic efficiency
The relative growth rate (RGR, mg g−1 DW day−1) was calculated by the following formula , where ΔW was the variation in wet weight (mg), WT1 was initial wet weight (g), and ΔT represented the culture time (28 days).
On day 28, a total of 15 branches of A. cf. fragilissima were haphazardly collected from each tank. Five branches were used for each measurement and subjected to dark for 30 min. The ratio of variable (Fv) to maximum (Fm) florescence (Fv/Fm), as an indicator of the maximum quantum efficiency of PSII, was measured by Hansatech FMS-2.
Pigment contents, phycocyanin, allophycocyanin, and phycoerythrin contents
Chlorophyll a (Chl-a) and carotenoids (Car) were calculated on day 28 (Lichtenthaler, 1987) and expressed as proportion of algal dry weight (mg g−1 DW).
Algal samples were subjected to freeze-thaw cycles and ultrasonic crushing on day 28. The contents of phycocyanin (PC), allophycocyanin (APC), and phycoerythrin (PE) were quantified spectrophotometrically in the supernatants at 565, 620, and 650 nm, respectively (Bermejo Roman et al., 2002), and expressed as proportion of algal dry weight (mg g−1 DW).
Net calcification rate and mineralogy analyses
The buoyant weighting technique was applied to calculate the net calcification rate (μmol CaCO3g−1DW day−1 ), by comparing the buoyant weights at the beginning and end of experiment (Peach et al., 2017). In addition, CaCO3 content (tissue mineral content) was determined at the end of experiment. Briefly, thalli were collected, washed, freeze dried, and weighed on day 28 (W1). Then, the samples were acidified with 5% HCl, lyophilized, and weighted (W2). CaCO3 content was calculated on the basis of the difference between W1 and W2 and expressed as the percent of algal dry weight.
Powder XRD and SEM-EDS were used to investigate the mineralogical responses of A. cf. fragilissima to conditional changes. Briefly, a portion of thalli were cleaned, freeze-dried, ground, and homogenized using a pestle and agate mortar. XRD measurements were performed in an angular range 2θ from 20° to 80° with a step scan of 0.02° using a Rigaku Ultima IV diffractometer with Cu Kα radiation. The tube conditions were 40 kV and 40 mA. Other algal samples were cleaned and dried using a critical point dryer (K850, Quorum technologies Ltd., Laughton, UK). After drying, the relative contents of elements were quantified using SEM (Regulus 8100, Hitachi, Japan)-EDS (Ultim Max 100, Oxford, UK). Meanwhile, the numbers of conceptacles per area on the algal surface were counted and expressed as conceptacles per square millimeter.
Statistical analyses
All statistical analyses were conducted using SPSS statistical software. Data were recorded as the mean ± standard deviation. Differences in the physiological parameters (i.e., RGR, pigment content, and net calcification rate) between different treatments were analyzed by one-way analysis of variance (ANOVA). The two-way ANOVA were performed to investigate the interaction effects between temperatures and light intensity on these physiological parameters in A. cf. fragilissima. When there were significant interactions, the Tukey post hoc test was used for multiple comparisons. P< 0.05 was considered statistically significant, whereas P< 0.01 was considered extremely statistically significant.
Results
Effects of increased temperature and light intensity on algal growth rate
The effects of temperature and light intensity on the photosynthesis and relative growth rate were investigated (Figure 1). As shown in Figure 1A, significant negative effects of increased light intensity or temperature on Fv/Fm were observed (F = 85.169, P = 0.003; F =31.427, P = 0.003, respectively; Table S1) although their interactive effects on Fv/Fm were not significant (F = 3.086, P = 0.155). When A. cf. fragilissima was exposed to high irradiance with 120 μmol photons m−2 s−1 (32°C, group A6), the value of Fv/Fm was lowest, which decreased by 56% compared with that of group A1 (26°C, 60 μmol photons m−2 s−1). Similarly, heat stress and light stress had both significant negative effects on the relative growth rate in Figure 1B (F = 646.024, P = 0.002; F = 394.775, P = 0.002, respectively; Table S1). The relative growth rate declined by approximately 50% at 32°C compared with that of 26°C group when the light intensity was 60 μmol photons m−2 s−1, although the thallus could grow at 32°C. The shift downward was more marked with the increasing light intensity (F = 1.517, P = 0.323; Table S1). When the light intensity was 120 μmol photons m−2 s−1, A. cf. fragilissima exhibited the lowest growth rate, which decreased from 12.6 (group A1) to 3.7 mg g−1 DW day−1 (group A6).
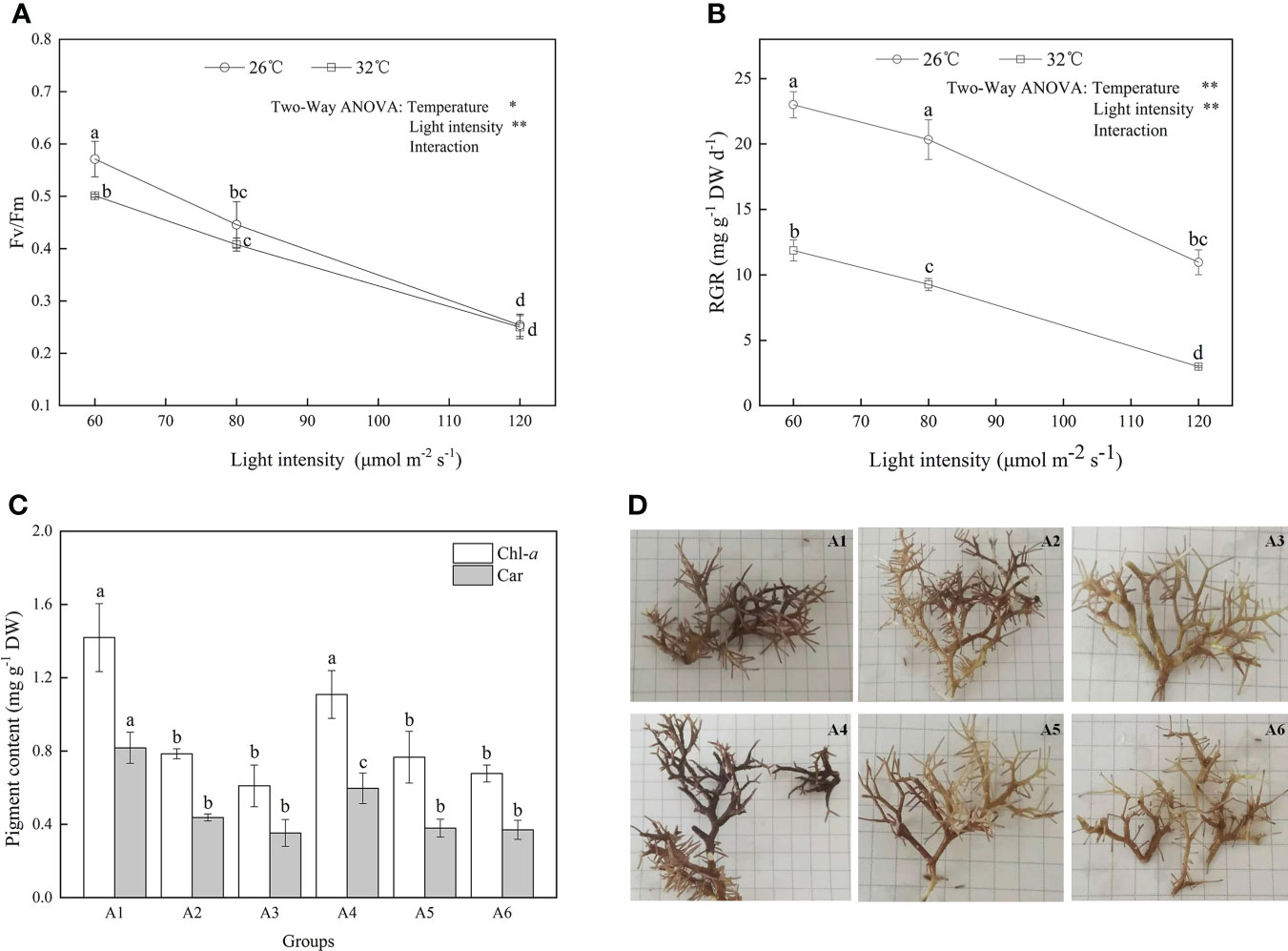
Figure 1 The (A) Fv/Fm, (B) relative growth rate, (C) pigment contents, and (D) morphological responses of A cf. fragilissima to increased temperature and light intensity (A1: 60 μmol photons m−2 s−1, 26°C; A2: 80 μmol photons m−2 s−1, 26°C; A3: 120 μmol photons m−2 s−1, 26°C; A4: 60 μmol photons m−2 s−1, 32°C; A5: 80 μmol photons m−2 s−1, 32°C; and A6: 120 μmol photons m−2 s−1, 32°C). Error bars correspond to the standard deviation with three independent replicates. abcd, Different letters implied significant differences (P < 0.05) for each parameter. *P < 0.05, **P < 0.01.
Effects of increased seawater temperature and light on algal pigment contents
As shown in Figure 1C, Chl-a and carotenoid contents significantly decreased at 32°C (F = 62.818, P = 0.016; F = 178.986, P = 0.006, respectively; Table 1). When light intensity increased from 60 to 80 μmol photons m−2 s−1, the contents of Chl-a and carotenoid were inhibited (Tukey test, P = 0.007 and 0.002, respectively); However, no significant differences were observed in these parameters between groups of 80 and 120 μmol photons m−2 s−1 (Tukey test, P = 0.283 and 0.423, respectively). Figure 1D showed that the difference in algal morphology was unapparent under low light condition when temperature increased from 26°C to 32°C. However, algal color gradually became white with the increase of light intensity (i.e., bleaching), which was consistent with the variation of Chl-a content. The algal morphology was further investigated using SEM. As shown in Figure 2, the number of conceptacles distributed on the algal surface was highest when light intensity was 60 μmol photons m−2 s−1 (groups A1 and A4; Figure S2). The number of conceptacles gradually decreased with the enhancement of light intensity (F = 265.750, P <0.001; Table S1). Tukey test showed that there were no significant differences between groups of 80 and 120 μmol photons m−2 s−1 (P = 0.685; Table S1). The conceptacles were hardly observed when light intensity was 120 μmol photons m−2 s−1 (groups A3 and A6).
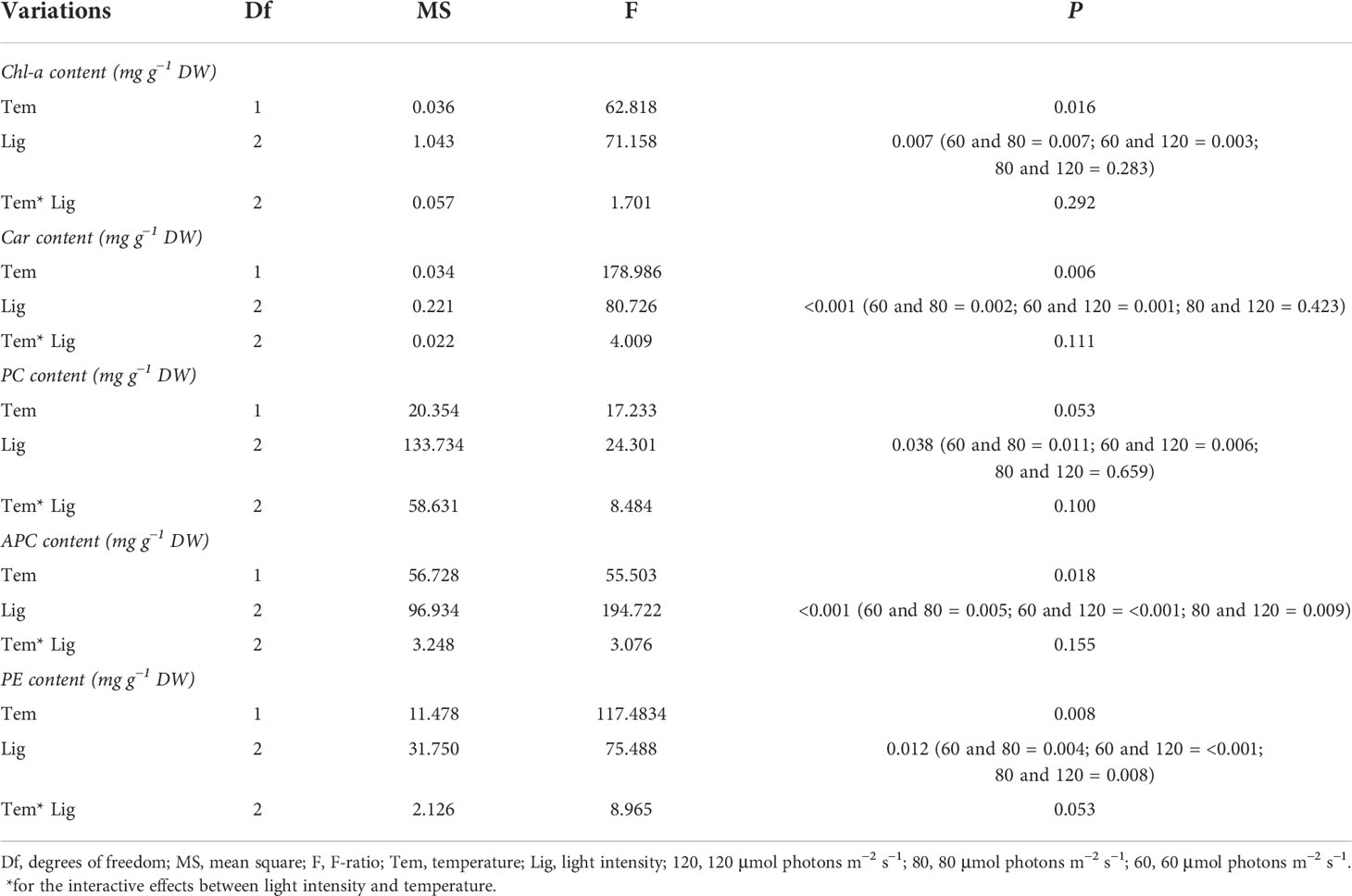
Table 1 Statistical analysis of Chl-a, Car, PC, APC, and PE of A. cf. fragilissima under different conditions.
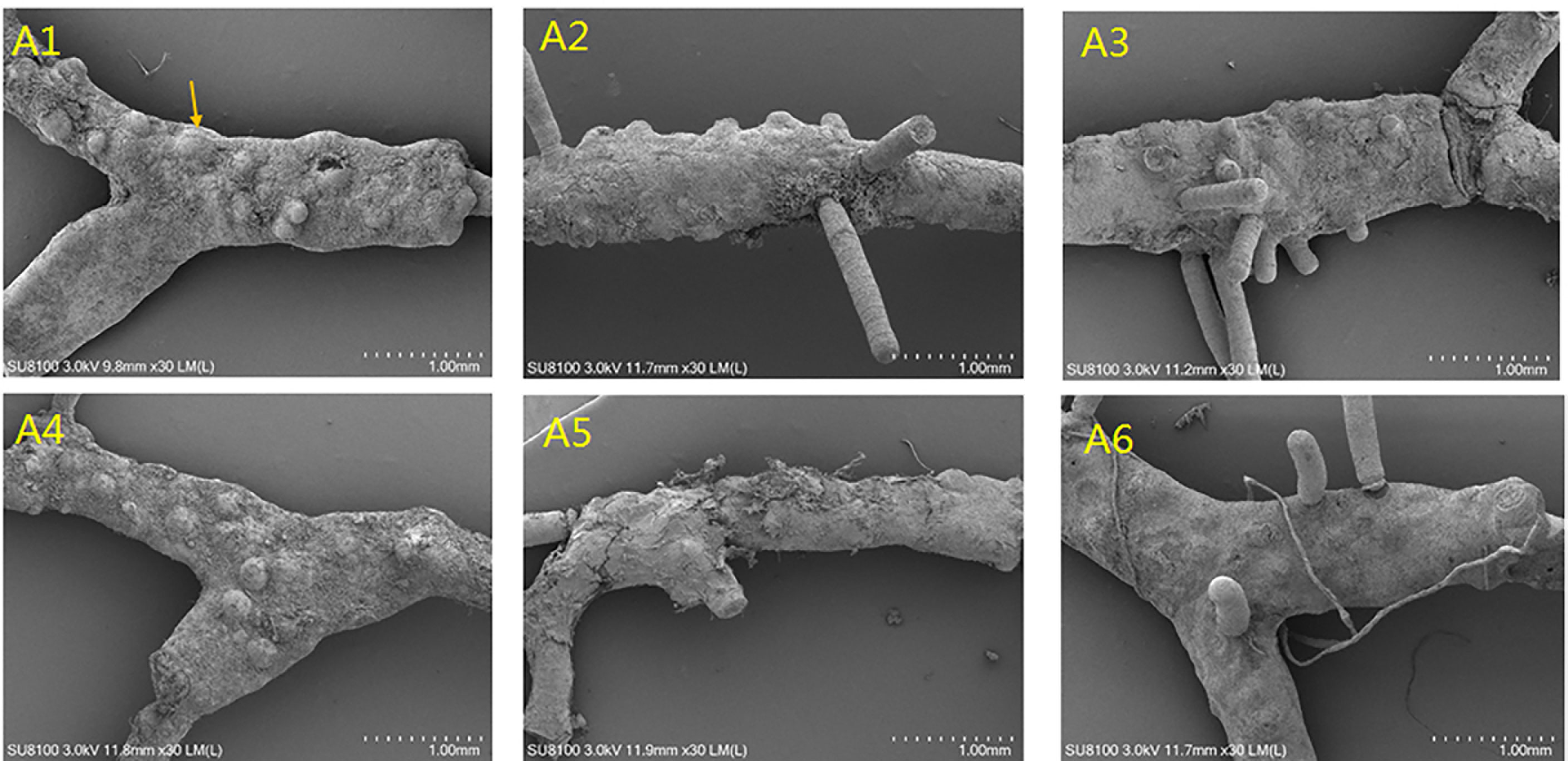
Figure 2 The effects of temperature and light intensity on algal surface by SEM (A1: 60 μmol photons m−2 s−1, 26°C; A2: 80 μmol photons m−2 s−1, 26°C; A3: 120 μmol photons m−2 s−1, 26°C; A4: 60 μmol photons m−2 s−1, 32°C; A5: 80 μmol photons m−2 s−1, 32°C; and A6: 120 μmol photons m−2 s−1, 32°C).
Figure 3 showed the contents of PE, PC, and APC among different treatments. Among these, APC content was highest, followed by PE, and PC in all treatments. APC content ranged from 1.93 to 12.70 mg g−1 DW. Heat stress significantly decreased APC content compared with 26°C groups (F = 55.503, P = 0.018; Table 1). Similarly, higher light intensity also inhibited APC accumulation (F = 194.722, P< 0.001, Table 1), resulting in a lowest value of 1.9 mg g−1 DW. As shown in Table 1, higher temperature and light intensity had both significant effects on PE content (F = 117.4834, P = 0.008; F = 75.488, P = 0.012, respectively); however, their interactive effects were not significant for PE content (P = 0.053; Table 1).
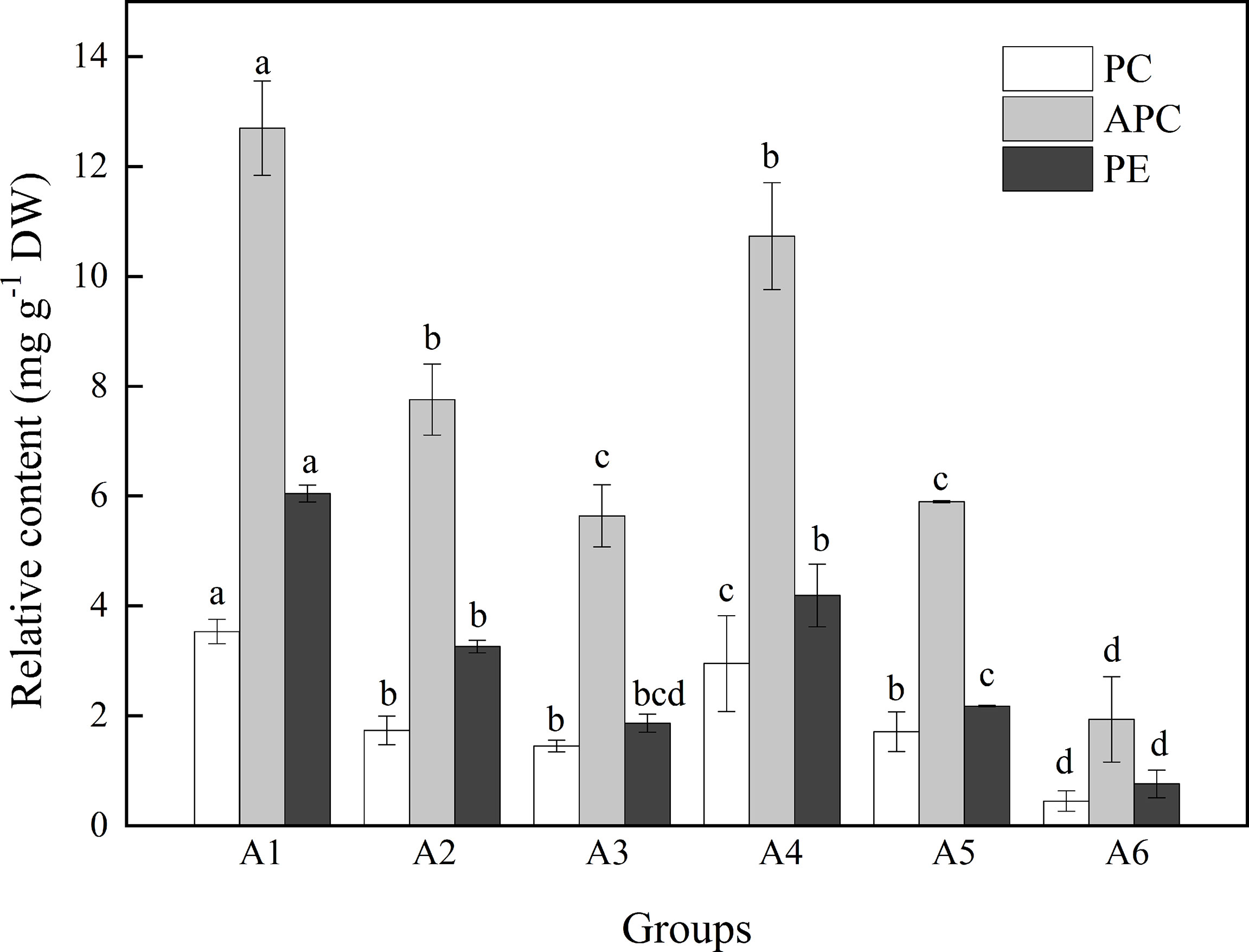
Figure 3 The contents (%) of PC, APC, and PE when A. cf. fragilissima was exposed to different conditions over 4 weeks (A1: 60 μmol photons m−2 s−1, 26°C; A2: 80 μmol photons m−2 s−1, 26°C; A3: 120 μmol photons m−2 s−1, 26°C; A4: 60 μmol photons m−2 s−1, 32°C; A5: 80 μmol photons m−2 s−1, 32°C; and A6: 120 μmol photons m−2 s−1, 32°C). Error bars correspond to the standard deviation with three independent replicates. abcd, Different letters implied significant differences (P < 0.05) for each parameter.
Effects of increased seawater temperature and light on algal calcification
As shown in Figure 4A, the tissue mineral content (i.e., CaCO3 content) accounted for approximately 92% of thallus under different cultivation conditions. Furthermore, it did not exhibit a remarkable change in CaCO3 content among treatments at the end of the experiment (P > 0.05). Figure 4B showed that there were obvious differences in the net calcification rate under heat stress or light stress (F = 14157.644, P < 0.001; F = 626.671, P < 0.001, respectively), whereas the interaction effects between light and temperature were not significant on the net calcification rate (F = 2.472, P = 0.200; Table S1). Compared with 60 μmol photons m−2 s−1, the net calcification rate did not significantly vary at 80 μmol photons m−2 s−1 (P = 0.203 and 0.095, respectively) but declined at 120 μmol photons m−2 s−1 (P = 0.003 and 0.002, respectively; Figure 4B). Furthermore, when seawater temperature was 32°C, the net calcification rate reached to the lowest value (10.92 μmol CaCO3g−1DW day−1 ; Figure 4B).
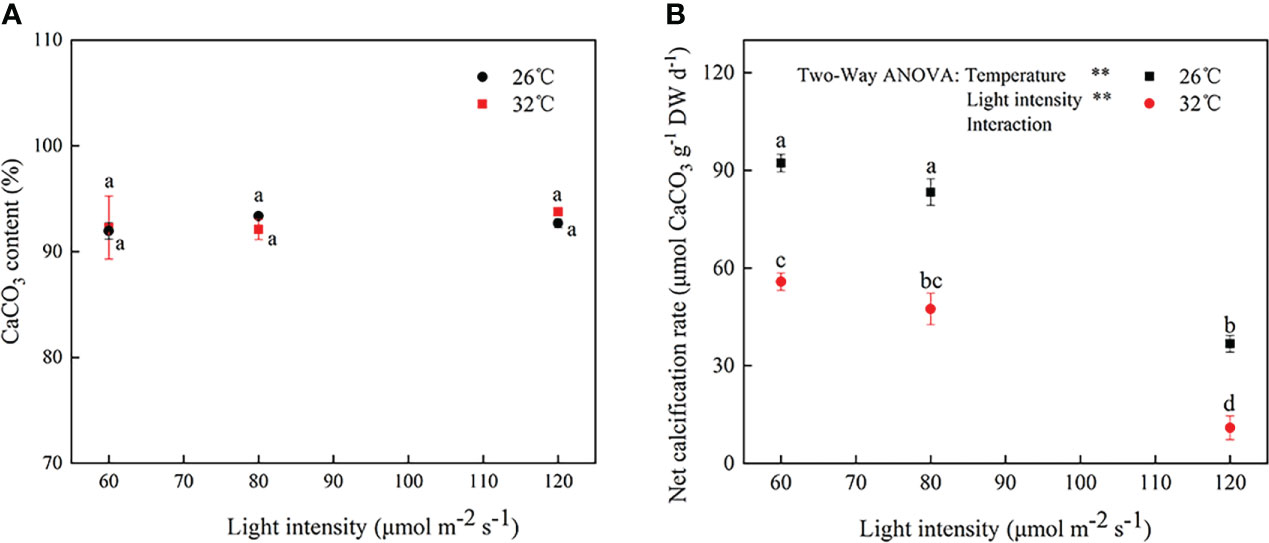
Figure 4 The (A) CaCO3 content and (B) net calcification rate in A cf. fragilissima after 4-week cultivation period under different conditions. Error bars correspond to the standard deviation with three independent replicates. abcd, Different letters implied significant differences (P < 0.05) for each parameter. **P < 0.01.
The mineral compositions were further investigated using XRD. Figure 5 showed a series of well-resolved diffraction peaks of the samples, including (104), (110), (113), (202), (018), and (116), which were in agreement with the power peaks obtained from the International Centre of Diffraction Data card (JCPDS card no. 05–0586.11). The result revealed that A. cf. fragilissima was mainly composed of carbonate calcite minerals. In addition, there was a higher calcite phase in group A1. However, there were no obvious differences in crystalline phase among groups.
SEM-EDS was applied to observe the algal morphology and elemental compositions. As shown in Figures 6 and 7, A. cf. fragilissima typically had calcite cell lining and cell infill. The mineral was mostly calcite in the form of CaCO3. The top surface view showed that cell facets of a relatively regular geometry and epibionts (such as diatoms) attached on the algal surface (Figure 6A). As shown in Figure 6B, the elemental compositions included C, O, Na, Mg, Al, P, S, and Ca. Ca content of pink surficial crust (pigmented layer) on the original thalli varied between 10.52% and 21.24%, which was significantly influenced by temperature (F = 11.484, P = 0.043), but it was not affected by light intensity (F = 5.781, P = 0.072; Table 2). Similarly, the unpigmented skeleton layer of thallus was mostly consisted of Ca-calcite (Figure 7). The content of Ca ranged from 15.19% to 22.96%. By statistical analysis, the Ca content of the skeleton layer was not significantly affected by temperature and light intensity (P = 0.268 and 0.054, respectively). In addition, low levels of magnesium calcite were observed in all groups, which were not affected by temperature or light intensity.
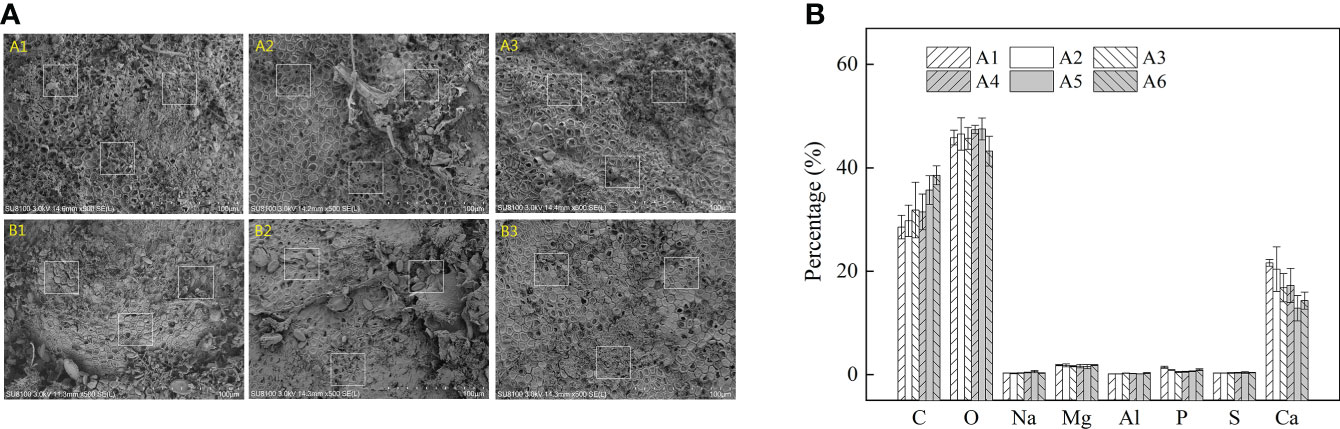
Figure 6 The (A) SEM pictures and (B) relative abundance (%) of main elements in the pink surficial crust/pigmented layer across temperature and light treatments for A cf. fragilissima (A1: 60 μmol photons m−2 s−1, 26°C; A2: 80 μmol photons m−2 s−1, 26°C; A3: 120 μmol photons m−2 s−1, 26°C; A4: 60 μmol photons m−2 s−1, 32°C; A5: 80 μmol photons m−2 s−1, 32°C; and A6: 120 μmol photons m−2 s−1, 32°C). Error bars correspond to the standard deviation with three independent replicates.
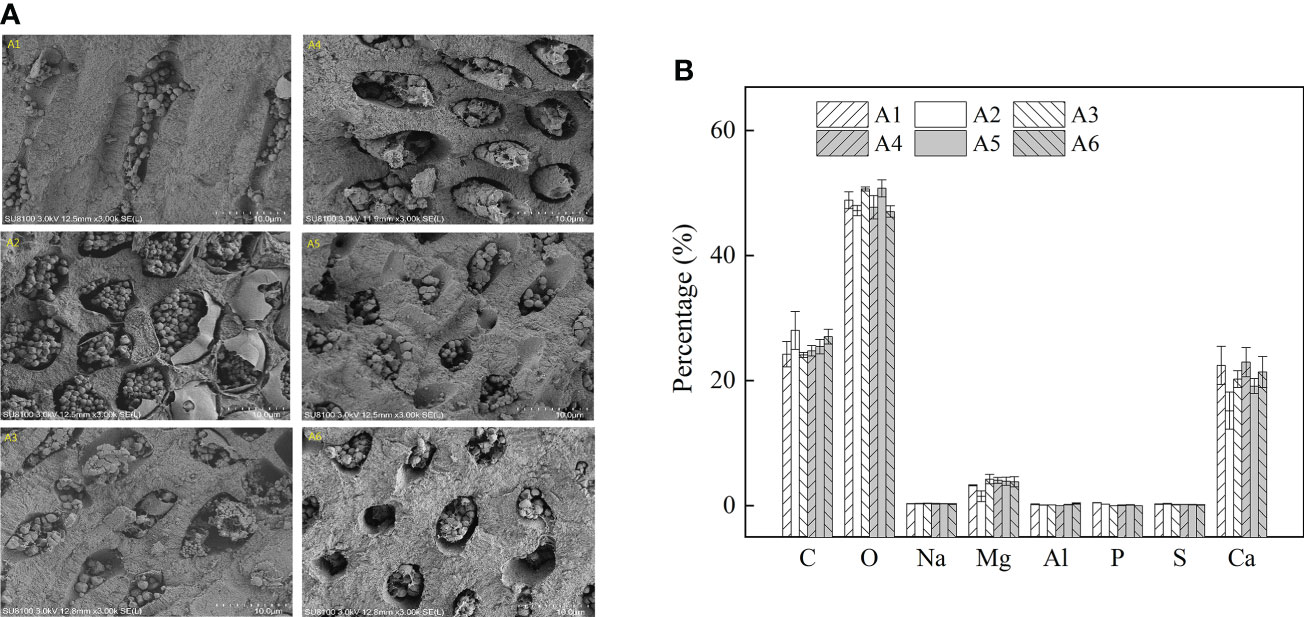
Figure 7 The (A) SEM pictures and (B) relative abundance (%) of main elements in the unpigmented skeletal layer under different temperature and light by SEM-EDS (A1: 60 μmol photons m−2 s−1, 26°C; A2: 80 μmol photons m−2 s−1, 26°C; A3: 120 μmol photons m−2 s−1, 26°C; A4: 60 μmol photons m−2 s−1, 32°C; A5: 80 μmol photons m−2 s−1, 32°C; and A6: 120 μmol photons m−2 s−1, 32°C). Error bars correspond to the standard deviation with three independent replicates.
Discussion
Coralline algae, marine calcifying macroalgae, are major contributors to primary productivity, CaCO3 sediment, and reef cementation (Nelson, 2009; Littler and Littler, 2013; Teichert et al., 2020). They are widely distributed from the tropics to polar latitudes and from intertidal zone to deeper water levels (Nelson, 2009; Schubert et al., 2020). Temperature and irradiance are considered as important factors that affect the algal physiology, growth, survival, abundance, and geographical distribution (Nejrup et al., 2013; Guy-Haim et al., 2016). For each algal species, generally, there is an optimal temperature and light range (Singh and Singh, 2015; Celis-Plá et al., 2020; Schmid et al., 2021). To date, the effects of temperature on coralline algae have been well investigated (Wilson et al., 2004; Diaz-Pulido et al., 2014; Ordoñez et al., 2017; Bach et al., 2017; Chan et al., 2020). However, the interaction effects between temperature and light on coralline algae remain unclear. Cornwall et al. (2022) have reported that coralline algae were more affected by light intensity compared with temperature. In our study, for A. cf. fragilissima, the optimal temperature and light intensity for calcification was 26°C–28°C (Yang et al., 2021b) and 60 μmol photons m−2 s−1, respectively. Furthermore, A. cf. fragilissima was more susceptible to high light stress than heat stress within 32°C, which was in agreement with previous studies (Yoshioka et al., 2020; Cornwall et al., 2022). Interestingly, increased light intensity and temperature resulted in the decrease of conceptacle numbers and the increase of algal bleaching in our study. Prathep et al. (2018) have observed that Halimeda become whiter when exposed to more than 1,200 μmol photons m−2 s−1 for mid-day hours in the field, which may contribute to protect algae from light stress. Similar phenomenon was observed in another study, in which C. officinalis exhibited relatively high bleaching at light stress (Kim et al., 2013). A. cf. fragilissima is generally found under fleshy brown or green macroalgae, which can protect it from strong sunshine; thus, it may be a low-light–adapted species. The enhancements in light or temperature that exceeded the physiological tolerance are likely to have strong influences for populations of A. cf. fragilissima.
The chlorophyll fluorescence parameter, Fv/Fm, is vital useful parameter to evaluate the eco-physiological responses to variations of environmental conditions. Huner et al. (2013) have reported that photoinhibition and photodamage occur when algae are exposed to high light intensity. Increasing temperature inhibited the net photosynthetic rate of Porolithon cf. onkodes (Page et al., 2021). In this study, Fv/Fm significantly decreased under thermal stress although the alga could survive within 4 weeks. The results combined with our previous data (Yang et al., 2021b), implying that A. cf. fragilissima could acclimate to thermal variation in short term by increasing antioxidant production; however, in the long-term exposure to environmental changes, this alga was seriously damaged and even bleached. In addition, Fv/Fm was significantly reduced at higher light intensity, revealing that the algal physiological responses to warming could be related to the degree of shade or sun stress experienced during their life history and depending on locality. Pigment is one of vital indicators of the stress conditions. In this study, A. cf. fragilissima exhibited a significant decrease in Chl-a content when light intensity was more than 80 μmol photons m−2 s−1, which coincided with the variation of Fv/Fm. Perhaps, the light level with 80 μmol photons m−2 s−1 was higher than saturating irradiance in A. cf. fragilissima. Similar phenomenon was documented in the previous literatures (Fortes and Lüning, 1980; Esteban et al., 2015). Fortes and Lüning (1980) have revealed that pigment contents of Saccharina latissima, Ulva lactuca, Porphyra umbilicalis, and Chondrus crispus significantly vary when exposed to light stress ranging from 30 to 70 μmol photons m−2 s−1. Similarly, Esteban et al. (2015) have reported that there are significant changes in Chl-a and PE levels when light intensities are between 30 and 60 μmol photons m−2 s−1. Our study demonstrated that Fv/Fm is a suitable parameter to evaluate the algal acclimation to environment stress.
Calcification is an important physiological process for calcified algae. Several studies have shown that calcification is a light-stimulated metabolic process (EI Haïkali et al., 2004; Guy-Haim et al., 2016), which can be influenced by light (Teichert and Freiwald, 2014). For example, Halimeda macroloba exhibited a significant decrease in the calcification rate when exposed to high light level of 1,200 μmol photons m−2 s−1 or low light level of 50 μmol photons m−2 s−1 (Prathep et al., 2018). Decalcification was even observed when algae were exposed to darkness or heat stresses (Kim et al., 2013). However, Williams et al. (2018) have thought that calcification process is metabolically driven because Clathromorphum compactum can calcify in complete darkness for 2 months. In our study, high light intensity significantly suppressed the net calcification rate of A. cf. fragilissima. However, the relationship between calcification process and light needs further research. In addition, Cornwall et al. (2019) have assessed the effects of warming on coralline algal calcification based on previous reports. They have found that there is a net negative impact of warming on algal calcification when temperature was 5.2°C higher than ambient condition. Similarly, in this present study, a negative impact was observed when temperature increased by 6°C. The data implied that A. cf. fragilissima could decrease the carbon fixation as a trade-off to living under light stress or heat stress.
The carbonate mineralogy of coralline algae is mainly composed of calcite, aragonite, and dolomite. Diaz-Pulido et al. (2014) have shown that ocean warming and acidification can induce the mineralogical changes, resulting in the enhancement of magnesium content and dolomite in P. onkodes, which may threat the structural integrity of reef ecosystems. However, mineralogical responses to high temperature and light intensity are unclear in coralline algae. Our study revealed that A. cf. fragilissima was mainly composed of carbonate calcite minerals, which was similar to the feature that documented previously in several coralline algae (Ries, 2010; Diaz-Pulido et al., 2014). In addition, increased temperature significantly decreased Ca content in algal pigmented layer; however, Ca content of the skeleton layer was not significantly affected by temperature and light intensity. Previous studies have suggested that the live, pigmented tissue layers have a vital role in modulating the mineralogical responses of coralline algae to environmental changes (Ries, 2006; Diaz-Pulido et al., 2014). Therefore, we speculated that the pigmented layer could limit mineralogical shifts of skeleton layer when exposed to short-term warming and cannot compensate for temperature influence.
Conclusions
This study showed that both high temperature and light intensity caused substantial changes in the physiological process of A. cf. fragilissima including major reductions in photosynthetic pigment, photosynthetic efficiency, conceptacle number, and growth. Furthermore, these physiological parameters were more strongly regulated by light. The lowest relative growth rate and algal bleaching were observed at light intensity of 120 μmol photons m−2 s−1. Meanwhile, after 4 weeks of exposure to high temperature, net calcification rate and Ca content in the pigment layer were inhibited, indicating that warming and irradiance will not only affect the growth, reproduction, and photosynthesis but also likely affect the calcification process. Our data also indicate the live pigmented layer may protect skeleton layer from mineral changes under short-term warming.
Data availability statement
The original contributions presented in the study are included in the article/Supplementary Material. Further inquiries can be directed to the corresponding authors.
Author contributions
FY conceived and designed the experiments. FY preformed the experiments and wrote the paper. ZW analyzed the data. LL and FY revised the paper, and performed final approval. All authors contributed to the article and approved the submitted version.
Funding
This research was supported by the National Natural Science Foundation of China (42176157), Key project of Chinese Academy of Sciences (2021000106), and Key Special Project for Introduced Talents Team of Southern Marine Science and Engineering Guangdong Laboratory (Guangzhou) (GML2019ZD0402).
Conflict of interest
The authors declare that the research was conducted in the absence of any commercial or financial relationships that could be construed as a potential conflict of interest.
Publisher’s note
All claims expressed in this article are solely those of the authors and do not necessarily represent those of their affiliated organizations, or those of the publisher, the editors and the reviewers. Any product that may be evaluated in this article, or claim that may be made by its manufacturer, is not guaranteed or endorsed by the publisher.
Supplementary material
The Supplementary Material for this article can be found online at: https://www.frontiersin.org/articles/10.3389/fmars.2022.922478/full#supplementary-material
References
Nelson W. A.2009 Calcified macroalgae–critical to coastal ecosystems and vulnerable to change: a review. Mar. Freshw. Res. 60, 787–801. doi: 10.1071/MF08335
Bach L. L., Freer J. J., Kamenos N. A. (2017). In situ response of tropical coralline algae to a novel thermal regime. Front. Mar. Sci. 4. doi: 10.3389/fmars.2017.00212
Bermejo Roman R., Alvarez-Pez J. M., Acien Fernandez F. G., Molina Grima E. (2002). Recovery of pure b-phycoerythrin from the microalga Porphyridium cruentum. J. Biotechnol. 93, 73–85. doi: 10.1016/S0168-1656(01)00385-6
Burdett H. L., Hatton A. D., Kamenos N. A. (2015). Coralline algae as a globally significant pool of marine dimethylated sulfur. Glob. Biogeochem. Cycle. 29, 1845–1853. doi: 10.1002/2015GB005274
Campbell J. E., Fisch J., Langdon C., Paul V. J. (2016). Increased temperature mitigates the effects of ocean acidification in calcified green algae (Halimeda spp.). Cor. Reef. 1, 357–368. doi: 10.1007/s00338-015-1377-9
Celis-Plá P. S. M., Moenne F., Rodríguez-Rojas F., Pardo D., Lavergne C., Moenne A., et al. (2020). Antarctic Intertidal macroalgae under predicted increased temperatures mediated by global climate change: Would they cope? Sci. Tot. Environ. 740, 140379. doi: 10.1016/j.scitotenv.2020.140379
Chan P. T. W., Halfar J., Adey W. H., Lebednik P. A., Steneck R., Norley C. J. D., et al. (2020). Recent density decline in wild-collected subarctic crustose coralline algae reveals climate change signature. Geology. 48, 226–230. doi: 10.1130/G46804.1
Comeau S., Carpenter R. C., Edmunds P. (2013). Effects of irradiance on the response of the coral Acropora pulchra and the calcifying alga Hydrolithon reinboldii to temperature elevation and ocean acidification. J. Exp. Mar. Biol. Ecol. 453, 28–35. doi: 10.1016/j.jembe.2013.12.013
Cornwall C. E., Diaz-Pulido G., Comeau S. (2019). Impacts of ocean warming on coralline algal calcification: meta-analysis, knowledge gaps, and key recommendations for future research. Front. Mar. Sci. 6. doi: 10.3389/fmars.2019.00186
Cornwall C. E., Harvey B. P., Comeau S., Cornwall D. L., Hall-Spencer J. M., Peña V., et al. (2022). Understanding coralline algal responses to ocean acidification: Meta-analysis and synthesis. Glob. Chang. Biol. 28, 362–374. doi: 10.1111/gcb.15899
Dean A. J., Steneck R. S., Tager D., Pandolf J. M. (2015). Distribution, abundance and diversity of crustose coralline algae on the great barrier reef. Cor. Reef. 34, 581–594. doi: 10.1007/s00338-015-1263-5
Diaz-Pulido G., Nash M. C., Anthony K. R. N., Bender D., Opdyke B. N., Reyes-Nivia C., et al. (2014). Greenhouse conditions induce mineralogical changes and dolomite accumulation in coralline algae on tropical reefs. Nat. Commun. 33105. doi: 10.1038/ncomms4310
Downs C. A., McDougall K. E., Woodley C. M., Fauth J. E., Richmond R. H., Kushmaro A., et al. (2013). Heat-stress and light-stress induce different cellular pathologies in the symbiotic dinoflagellate during coral bleaching. PloS One 8, e77173. doi: 10.1371/journal.pone.0077173
EI Haïkali B., Bensoussan N., Romano J. C., Bousquet. V. (2004). Estimation of photosynthesis and calcification rates of Corallina elongata Ellis and solander 1786, by measurements of dissolved oxygen, pH and total alkalinity. Sci. Mar. 68, 45–56. doi: 10.3989/scimar.2004.68n145
Esteban R., Barrutia O., Artetxe U., Fernandez-Marin B., Hernandez A., Garcia-Plazaola J. I. (2015). Internal and external factors affecting photosynthetic pigment composition in plants: a meta-analytical approach. New. Phytol. 206, 268–280. doi: 10.1111/nph.13186
Fortes M. D., Lüning K. (1980). Growth-rates of north-sea macroalgae in relation to temperature, irradiance and photoperiod helgol. Meeresunters. 34, 15–29. doi: 10.1007/BF01983538
Gomez-Lemos L. A., Diaz-Pulido G. (2017). Crustose coralline algae and associated microbial biofilms deter seaweed settlement on coral reefs. Cor. Reef. 36, 453–462. doi: 10.1007/s00338-017-1549-x
Guy-Haim T., Silverman J., Raddatz S., Wahl M., Israel A., Rilov G. (2016). The carbon turnover response to thermal stress of a dominant coralline alga on the fast warming Levant coast. Limnol. Oceanogr. 61, 1120–1133. doi: 10.1002/lno.10279
Huner N. P. A., Öquist G., Melis A. (2003). “Photostasis in plants, green algae and cyanobacteria: The role of light harvesting antenna complexes,” in Light-harvesting antennas in photosynthesis. advances in photosynthesis and respiration. Eds. Green B. R., Parson W. W. (Dordrecht: Springer), 13. doi: 10.1007/978-94-017-2087-8_14
Huner N. P. A., Bode R., Dahal K., Busch F. A., Possmayer M., Szyszka B. (2013). “Shedding some light on cold acclimation, cold adaptation, and phenotypic plasticity. Botany. 91127–136. doi: 10.1139/cjb-2012-0174
Jørgensbye H. I. Q., Halfar J. (2017). Overview of coralline red algal crusts and rhodolith beds (Corallinales, rhodophyta) and their possible ecological importance in Greenland. Pol. Biol. 40, 517–531. doi: 10.1007/s00300-016-1975-1
Jiang L., Sun Y. F., Zhang Y. Y., Zhou G. W., Li X. B., McCook L. J., et al. (2017). Impact of diurnal temperature fluctuations on larval settlement and growth of the reef coral Pocillopora damicornis. Biogeosciences. 14, 5741–5752. doi: 10.5194/bg-14-5741-2017
Kim J. H., Lam S. M. N., Kim K. Y. (2013). Photoacclimation strategies of the temperate coralline alga Corallina officinalis: a perspective on photosynthesis, calcification, photosynthetic pigment contents and growth. Algae. 28, 355–363. doi: 10.4490/algae.2013.28.4.355
Levy O., Marangoni L. F. D. B., Benichou J. I. C., Rottier C., Béraud E., Grover R., et al. (2020). Artificial light at night (ALAN) alters the physiology and biochemistry of symbiotic reef building corals. Environ. pollut. 266, 114987. doi: 10.1016/j.envpol.2020.114987
Lichtenthaler H. K. (1987). Chlorophyll and carotenoids: pigments of photosynthetic biomembranes. Method. Enzymol. 148, 331–382. doi: 10.1016/0076-6879(87)48036-1
Littler M. M., Littler D. S. (2013). The nature of crustose coralline algae and their interactions on reefs. Smith. Contrib. Mar. Sci. 39, 199–212. http://hdl.handle.net/10088/21634
Martone P. T., Alyono M., Stites S. (2010). Bleaching of an intertidal coralline alga: untangling the effects of light, temperature, and desiccation. Mar. Ecol. Prog. Ser. 416, 57–67. doi: 10.3354/meps08782
McCoy S. J., Kamenos N. A. (2015). Coralline algae (Rhodophyta) in a changing world: integrating ecological, physiological, and geochemical responses to global change. J. Phycol. 51, 6–24. doi: 10.1111/jpy.12262
Nejrup L. B., Staehr P. A., Thomsen M. S. (2013). Temperature-and light-dependent growth and metabolism of the invasive red algae Gracilaria vermiculophylla-a comparison with two native macroalgae. Eur. J. Phycol. 48, 295–308. doi: 10.1080/09670262.2013.830778
Ordoñez A., Kennedy E. V., Diaz-Pulido G. (2017). Reduced spore germination explains sensitivity of reef-building algae to climate change stressors. PloS One 12, e0189122. doi: 10.1371/journal.pone.0189122
Page T. M., Bergstrom E., Diaz-Pulido G. (2021). Acclimation history of elevated temperature reduces the tolerance of coralline algae to additional acute thermal stress. Front. Mar. Sci. 07. doi: 10.3389/fmars.2021.660196
Peach K. E., Koch M. S., Blackwelder P. L., Manfrino C. (2017). Calcification and photophysiology responses to elevated pCO2 in six Halimeda species from contrasting irradiance environments on little Cayman island reefs. J. Exp. Mar. Biol. Ecol. 486, 114–126. doi: 10.1016/j.jembe.2016.09.008
Pörtner H. O., Scholes R. J., Agard J., Archer E., Arneth A., Bai X. (2021). IPBES-IPCC co-sponsored workshop report synopsis on biodiversity and climate changee. IPBES and IPCC. doi: 10.5281/zenodo.4782538
Prathep A., Kaewsrikhaw R., Mayakun J., Darakrai A. (2018). The effects of light intensity and temperature on the calcification rate of Halimeda macroloba. J. Appl. Phycol. 30, 3405–3412. doi: 10.1007/s10811-018-1534-y
Rendina F., Bouchet P. J., Appolloni L., Russo G. F., Sandulli R., Kolzenburg R., et al. (2019). Physiological response of the coralline alga Corallina officinalis l. @ to both predicted long-term increases in temperature and short-term heatwave events. Mar. Environ. Res. 150, 104764. doi: 10.1016/j.marenvres.2019.104764
Ries J. B. (2006). Mg fractionation in crustose coralline algae: geochemical, biological, and sedimentological implications of secular variation in the Mg/Ca ratio of seawater. Geochim. Cosmochim. Acta 70, 891–900. doi: 10.1016/j.gca.2005.10.025
Ries J. B. (2010). Review: geological and experimental evidence for secular variation in seawater Mg/Ca (calcite-aragonite seas) and its effects on marine biological calcification. Biogeosciences. 7, 2795–2849. doi: 10.5194/bg-7-2795-2010
Rosic N., Rémond C., Mello-Athayde M. A. (2020). Differential impact of heat stress on reef-building corals under different light conditions. Mar. Environ. Res. 158, 104947. doi: 10.1016/j.marenvres.2020.104947
Sañé E., Chiocci F. L., Basso D., Martorelli E. (2016). Environmental factors controlling the distribution of rhodoliths: an integrated study based on seafloor sampling, ROV and side scan sonar data, offshore the W-pontine archipelago. Cont. Shelf. Res. 129, 10–22. doi: 10.1016/j.csr.2016.09.003
Sarkar S. (2017). Ecology of coralline red algae and their fossil evidences from India. Thalassas. 33, 15–28. doi: 10.1007/s41208-016-0017-7
Schmid M., Guihéneuf F., Nitschke U., Stengel D. B. (2021). Acclimation potential and biochemical response of four temperate macroalgae to light and future seasonal temperature scenarios. Algal. Res. 54, 102190. doi: 10.1016/j.algal.2021.102190
Schubert N., Schoenrock K. M., Aguirre J., Kamenos N. A., Silva J., Horta P. A., et al. (2020). Editorial: Coralline algae: Globally distributed ecosystem engineers. Front. Mar. Sci. 25. doi: 10.3389/fmars.2020.00352
Singh S. P., Singh P. (2015). Effect of temperature and light on the growth of algae species: A review. Renew. Sust. Energ. Rev. 50, 431–444. doi: 10.1007/BF00350071
Steneck R. S. (1986). The ecology of coralline algal crusts-convergent patterns and adaptative strategies. Annu. Rev. Ecol. Syst. 17, 273–303. doi: 10.1146/annurev.es.17.110186.001421
Suggett D. J., Dong L. F., Lawson T., Lawrenz E., Torres L., Smith D. J. (2013). Light availability determines susceptibility of reef building corals to ocean acidification. J.Cor. Reef. 32, 327–337. doi: 10.1007/s00338-012-0996-7
Sully S., Burkepile D. E., Donovan M. K., Hodgson G., van Woesik R. (2019). A global analysis of coral bleaching over the past two decades. Nat. Commun. 101264. doi: 10.1038/s41467-019-09238-2
Tanaka Y., Suzuki A., Sakai K. (2017). Effects of elevated seawater temperature and phosphate enrichment on the crustose coralline alga Porolithon onkodes (Rhodophyta). Phycol. Res. 65, 51–57. doi: 10.1111/pre.12152
Teichert S., Freiwald A. (2014). Polar coralline algal CaCO3-production rates correspond to intensity and duration of the solar radiation. Biogeosciences. 11, 833–842. doi: 10.5194/bg-11-833-2014 2014
Teichert S., Steinbauer M., Kiessling W. (2020). A possible link between coral reef success, crustose coralline algae and the evolution of herbivory. Sci. Rep. 10, 17748. doi: 10.1038/s41598-020-73900-9
Van der Heijden L. H., Kamenos N. A. (2015). Reviews and syntheses: Calculating the global contribution of coralline algae to total carbon burial. Biogeosciences. 12, 6429–6441. doi: 10.5194/bg-12-6429-2015
Vásquez-Elizondo R., Enríquez S. (2016). Coralline algal physiology is more adversely affected by elevated temperature than reduced pH. Sci. Rep. 6, 19030. doi: 10.1038/srep19030
Wai M. K. (2018). Morphotaxonomy, culture studies and phytogeographical distribution of Amphiroa fragilissima (Linnaeus) lamouroux (Corallinales, rhodophyta) from Myanmar. J. Aquac. Mar. Biol. 7, 142–150. doi: 10.15406/jamb.2018.07.00201
Williams S., Adey W., Halfar J., Kronz A., Gagnon P., Bélanger D., et al. (2018). Effects of light and temperature on mg uptake, growth, and calcification in the proxy climate archive Clathromorphum compactum. Biogeosciences. 15, 5745–5759. doi: 10.5194/bg-15-5745-2018
Williams E. A., Craigie A., Yeates A., Degnan S. M. (2008). Articulated coralline algae of the genus Amphiroa are highly effective natural inducers of settlement in the tropical abalone Haliotis asinine. Biol. Bull. 215, 98–107. doi: 10.2307/25470687
Wilson S., Blake C., Berges J. A., Maggs C. A. (2004). Environmental tolerances of free-living coralline algae (maerl): Implications for European marine conservation. Biol. Conserv. 120, 279–289. doi: 10.1016/j.biocon.2004.03.001
Yang F. F., Mo J. H., Wei Z. L., Long L. J. (2020). Calcified macroalgae and their bacterial community in relation to larval settlement and metamorphosis of reef-building coral Pocillopora damicornisFEMS Microbiol. Ecol. 97, fiaa215. doi: 10.1093/femsec/fiaa215
Yang F. F., Wei Z. L., Long L. J. (2021a). Macroalgal calcification and the effects of ocean acidification and global warming. Mar. Freshw. Res. 72, 1697–1710. doi: 10.1071/MF20316
Yang F. F., Wei Z. L., Long L. J. (2021b). Transcriptomic and physiological responses of the tropical reef calcified macroalga Amphiroa fragilissima to elevated temperature. J. Phycol. 57, 1254–1265. doi: 10.1111/jpy.13158
Keywords: coralline algae, Amphiroa cf. fragilissima, warming, irradiance, growth, calcification, mineralogical composition
Citation: Yang F, Wei Z and Long L (2022) Impacts of ocean warming on a reef-building coralline alga Amphiroa cf. fragilissima under high irradiance. Front. Mar. Sci. 9:922478. doi: 10.3389/fmars.2022.922478
Received: 18 April 2022; Accepted: 29 July 2022;
Published: 23 August 2022.
Edited by:
Peng Jin, University of Guangzhou, ChinaReviewed by:
Futian Li, Jiangsu Ocean University, ChinaAmit Ghosh, Birbal Sahni Institute of Palaeobotany, India
Marco Munari, Anton Dohrn Zoological Station, Italy
Copyright © 2022 Yang, Wei and Long. This is an open-access article distributed under the terms of the Creative Commons Attribution License (CC BY). The use, distribution or reproduction in other forums is permitted, provided the original author(s) and the copyright owner(s) are credited and that the original publication in this journal is cited, in accordance with accepted academic practice. No use, distribution or reproduction is permitted which does not comply with these terms.
*Correspondence: Fangfang Yang, ycuyang@scsio.ac.cn; Lijuan Long, longlj@scsio.ac.cn