Physiological Response of Shallow-Water Hard Coral Acropora digitifera to Heat Stress via Fatty Acid Composition
- 1Institute of Oceanography and Environment, Universiti Malaysia Terengganu, Kuala Terengganu, Malaysia
- 2Faculty of Science and Marine Environment, Universiti Malaysia Terengganu, Kuala Terengganu, Malaysia
Heat stress disturbs the mutualistic relationship between the hard corals and the symbiotic algae, which cause coral bleaching. A wide array of biochemical parameters is used to demonstrate the phenomenon. This study exposed a shallow-water hard coral, Acropora digitifera, to a series of elevated temperatures over time while the interaction between Symbiodiniaceae (SD) density, antioxidants activities, fatty acid (FA) composition, and putative FA health indicators was evaluated. Heat stress caused a substantial loss in SD densities, consequently regulated the antioxidant activities and caused significant changes in FA composition. There was a lack of evidence showing A. digitifera experienced oxidative stress; nonetheless, a significant decrease of monounsaturated fatty acid as (MUFA) and polyunsaturated fatty acid (PUFA) during the thermally induced experiment demonstrated that corals utilize their unsaturated FA as a final barrier or as a repair system against oxidative damage once the antioxidant enzyme cannot cope with stress condition. The lower ratio of putative FA health indicators [i.e., n-3 LC:n-6 LC, eicosapentaenoic acid (EPA):arachidonic acid (ARA), and docosahexaenoic acid (DHA):ARA] characterized an unhealthy coral. The loss of SD density was significantly correlated with certain PUFA markers [i.e., linolenic acid (18:3n6), 20:5n3, and 22:6n3] and putative FA health indicator (i.e., n-3 LC:n-6 LC, EPA:ARA, and DHA:ARA). These notably imply that the FA linked with the symbiont can be a potential health indicator for assessing the effect of the environmental stressor on coral. This study also revealed the regulation of FAs during stress conditions, especially when heterotrophic feeding is limited. Future studies on FA profiles toward antagonistic or synergistic effects will offer a better understanding of the nature of this relationship under a harsh climate.
Introduction
Coral reefs are declining worldwide subjected to natural and anthropogenic disturbances (Baker et al., 2008; De’ath et al., 2012; Cziesielski et al., 2019). During the stressful environmental condition, such as extremes in temperature, oxygen, salinity, and nutrient supply, the physiology of corals changes and leads to the disappearance of symbiotic algae or known as coral bleaching (Baird et al., 2008; Apprill, 2020). The cellular mechanism underlying the bleaching is tightly coupled with the accumulation of reactive oxygen species (ROS), which damages the D1 protein of photosystem II (PSII) in the thylakoid membrane of the symbiotic algae (Downs et al., 2002; Bhagooli and Hidaka, 2004; Lesser, 2006; Baird et al., 2008; Takahashi and Murata, 2008). Extended photooxidative damage and consequential photoinhibition of PSII should overwhelm the antioxidant system on both partners and should increase the cellular damage such as heat shock proteins and other danger-associated molecular patterns, which later suggesting the breakdown of symbiosis (Lesser, 1997; Downs et al., 2002; Takahashi and Murata, 2008; Weis, 2008; Palmer, 2018; Mansfield and Gilmore, 2019). Studies have shown that oxidative stress is primarily originated from the symbiotic algae and causes tissue damage to the host (Nesa and Hidaka, 2009; Yakovleva et al., 2009; Cunning and Baker, 2012). The production of ROS occurs in the chloroplast by the process linked with PSI- and PSII-catalyzed electron transfer, leading to the production of superoxide radical (O2–) and hydrogen peroxide (H2O2), which diffuses to the coral cytoplasm but it can be counterbalanced by enzymatic and non-enzymatic antioxidants from coral (Downs et al., 2002). The cellular antioxidant enzymes such as superoxide dismutases (SODs), peroxidases, catalases, and mycosporine-like amino acids function as scavengers to ROS and act as the primary defense against oxidative stress (Cook and Davy, 2001; Biel et al., 2007; Baird et al., 2008). However, under prolonged and severe stress, the antioxidant system might be unable to cope with the excessive production of ROS and cause oxidative damage to the cells (Baird et al., 2008). Due to this, the coral will regulate the secondary defense or repair system to fight against endogenous stress.
It is known that marine organisms regulate their fatty acid (FA) and sterol composition as a defense mechanism from the influence of changing temperature, pressure, or lipid peroxidation (Parrish, 2013). Field and experimental studies demonstrated that stress-related condition induces the FA composition, resulting in low FA composition (Bachok et al., 2006; Imbs et al., 2014; Rodríguez-Troncoso et al., 2016). Reduction in FA composition is directly coupled with their photosynthetic symbionts (Papina et al., 2003; Imbs et al., 2010b). The loss of the symbiotic algae during coral bleaching alters the FA composition in the coral, especially the polyunsaturated fatty acid (PUFA), contents (Bachok et al., 2006; Imbs and Yakovleva, 2012; Rodríguez-Troncoso et al., 2016). This is because some of the PUFAs were translocated from the symbiont to the host (Imbs and Yakovleva, 2012).
The PUFAs play an important role as a constituent of the phospholipids of all cell membranes (Calder, 2010). In general, some of the PUFAs mainly originated from primary producers and passed up to the animals via the food chain (Monroig et al., 2013). In a clinical test, the consumption of PUFA such as n-3 or known as omega-3 PUFAs is important for human health due to their capability of partly inhibiting many aspects of inflammation (Calder, 2010). In corals, the PUFAs of the n-3 series are mainly originated from symbiotic algae or external food sources via heterotrophy because the animal cannot produce these essential PUFAs (Papina et al., 2003; Imbs et al., 2010b). Experimental work by Imbs and Yakovleva (2012) found that PUFAs such as 18:3n6 and stearidonic acid (18:4n3) in Montipora digitata and Acropora intermedia were significantly reduced under thermal stress conditions. While the reduction of those PUFAs is associated with the loss of symbiotic algae, PUFA also plays an important role in the regulation of stress conditions (Betteridge, 2000). It is suggested that the depletion of PUFA composition after the loss of symbiont can be associated with oxidative stress (Imbs and Yakovleva, 2012; Rodríguez-Troncoso et al., 2016). When oxidative defenses are overwhelmed, the excess radicals will accumulate and react with the double bond in unsaturated FAs, especially in PUFAs that are susceptible to peroxidation (Catalá, 2010). In marine organisms, both n-3 and n-6 PUFAs such as eicosapentaenoic acid (EPA; 20:5n3), docosahexaenoic acid (DHA; 22:6n3), and arachidonic acid (ARA; 20:4n6) play an important role against oxidative stress (Okuyama et al., 2008; Parrish, 2013); hence, it has been used as coral health indicator (Rocker et al., 2019; Kim et al., 2021). Meanwhile, coral increased the production of saturated fatty acid (SAFA) to maintain the rigidity of their biological membrane (Imbs and Yakovleva, 2012) as the SAFAs are less reactive and more stable than unsaturated FAs (Ratnayake and Galli, 2009). The application of FAs (Bachok et al., 2006; Imbs and Yakovleva, 2012; Rodríguez-Troncoso et al., 2016) and antioxidant activities (Yakovleva et al., 2004; 2009; Teixeira et al., 2013) to evaluate the stress response in corals is well established. However, the interaction between these parameters during stress conditions is not well described and experimentally tested. For that reason, we examined the composition of FAs in the stress-induced experiment via heat stress to increase the understanding of the role of FAs during stress conditions. The aims of this study were (1) to investigate the changes in the composition of FAs, Symbiodiniaceae (SD) density, and antioxidant activities of coral species, Acropora digitifera, under elevated temperature and (2) to examine the relationship between the SD density with FA markers and health indicator. This study hypothesized that the environmental stressor such as heat stress significantly affects the SD density, antioxidant activities, and FA composition in hard corals consequently resulting in the potential role of specific FAs as bioindicators.
Materials and Methods
Sample Collection
Colonies of shallow-water coral, A. digitifera, were collected in Pantai Pasir Tenggara (5°36′42.15″N, 103°3′32.44″E), Pulau Bidong, Terengganu, Malaysia (Figure 1A) where the species can be found abundantly in the area (Figure 1B). The samples were collected using a hammer and chisel (Figure 1C). The range of in situ water temperature was from 26.5 to 28.0∘C, with a salinity of 32.0 ± 0.5 ppt. The intensity of light penetrates the seawater was measured using a light meter (LI-COR LI-250A) with a measurement ranging from 150 to 200 μmol s–1 m–2. The selection of healthy samples of A. digitifera was performed using Coral Color Reference Card developed by Siebeck et al. (2006) as a reference, and all samples were collected from a non-shaded area. A total of three different colonies were collected at a distance of at least 5 m to minimize the probability of genetic clones, and each colony was separated into four sub-colonies that consist of at least eight coral fragments. Relatively, the samples were taken at the same depth around 3–4 m during high tide. Samples were separately placed in a labeled transparent plastic bag filled with in situ seawater (Figure 1D) and kept in a container with ice and seawater before reaching land. Back on land, samples were washed to remove any unwanted organisms (Figure 1E) and transferred into the designated tanks.
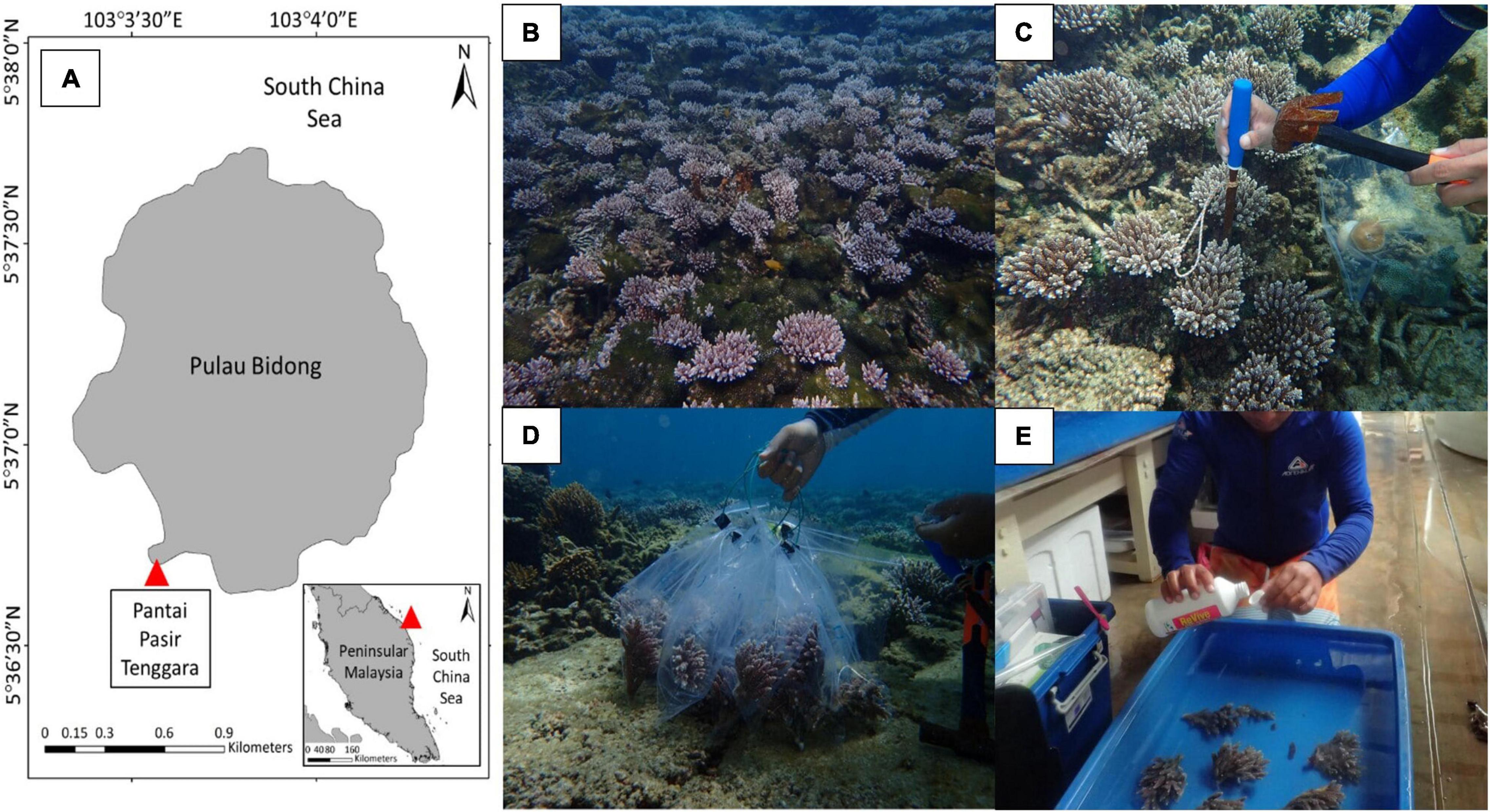
Figure 1. Map shows the location of sampling sites in Pantai Pasir Tenggara, Pulau Bidong located in the east coast of Peninsular Malaysia (A). Colonies of Acropora digitifera in Pantai Pasir Tenggara, Pulau Bidong can be found abundantly in the area (B). A diver using a hammer and a chisel for collecting samples (C) and keeps them inside a transparent bag (D). Back on land, samples were washed using ReVive Coral Cleaner solution and rinsed with clean seawater before placing the samples into the tanks (E).
Experimental Tank Preparation
The experiment was conducted in an indoor hatchery using two rectangular fiberglass tanks separated into control tank (CT) and exposure tank (ET). Each tank was equipped with a full-spectrum artificial light (Shark G90 195W LED Casing Lamp) to consistently illuminate both tanks. To provide continuous water flow inside the tank, wavemakers (SOBO aquarium super wavemaker WP-300M) were installed to provide vigorous aeration and kept the water well mixed throughout the experimental period. The temperature of treated seawater (32 ± 1.0 ppt) was acclimatized at 27 ± 1.0∘C using an aquarium chiller (Hailea HX-90A 0.5 hp) for 30 days preceding sample collection day. A schematic diagram of the experimental design is shown in Figure 2.
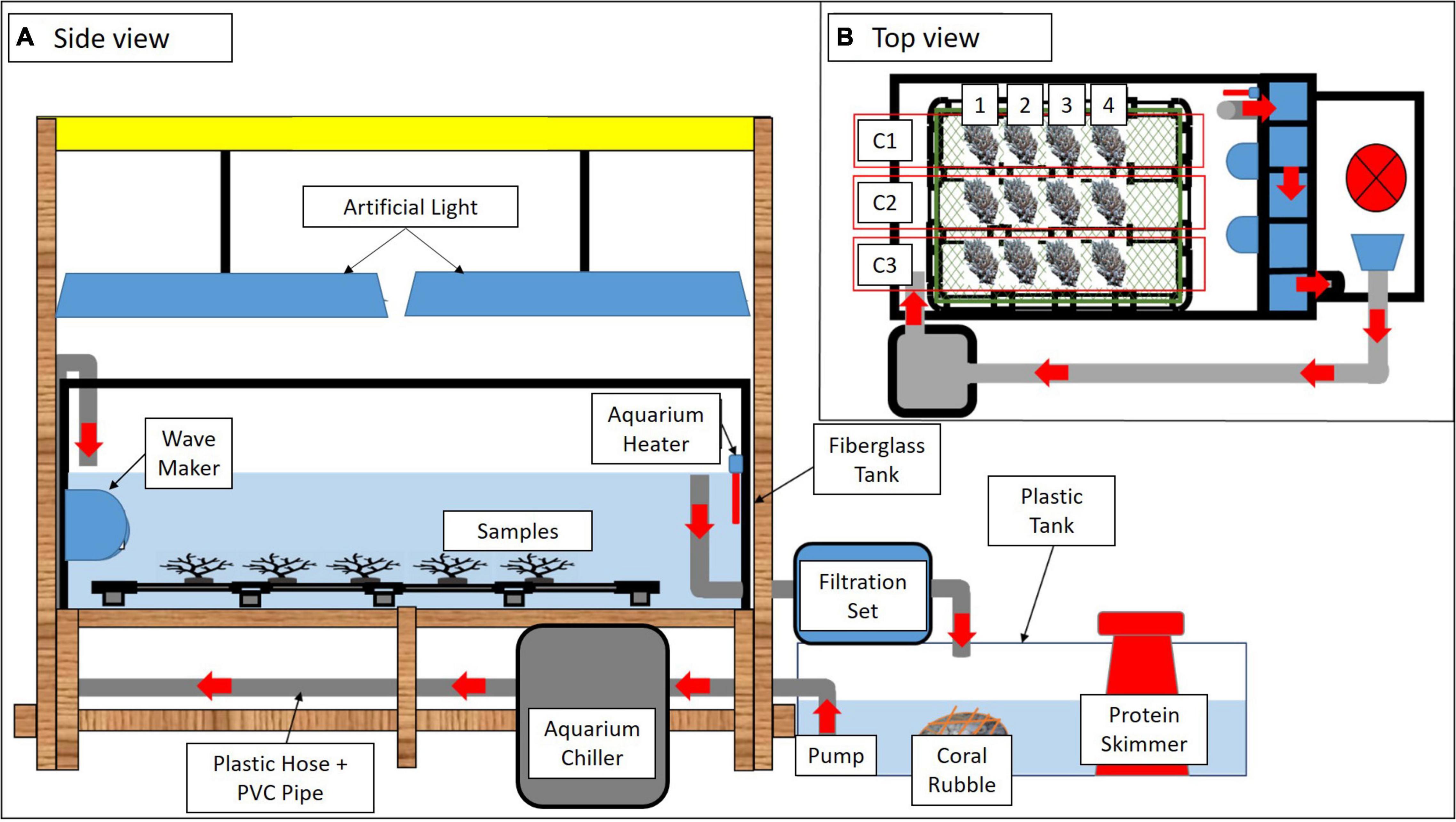
Figure 2. A schematic diagram of the experimental tank set up in different views (A,B). Red arrows indicate water flow. Three colonies of A. digitifera (C1, C2, and C3) separated into four sub-colonies (1–4) were arranged in each tank (B).
Maintenance of Live Acropora digitifera
Three colonies of A. digitifera with 5- to 6-cm-long branches were broken into sub-colonies. Each sub-colony comprised 8–10 branches and was transferred into the tanks accordingly. Each of the sub-colonies was placed on a cylindrical polyvinyl chloride pipe to support the samples in an upright position. In total, each tank contained 12 sub-colonies. The coral colonies were acclimatized for 10 days without feeding. The temperature and salinity of the seawater were maintained at relatively similar to in situ measurements (temperature = 27 ± 1.0∘C, salinity = 32 ± 1.0 ppm). Both tanks were illuminated with artificial light (±150 μmol photons m–2 s–1) on a 12:12-h light/dark cycle. During the acclimatization, all samples exhibited active polyp extensions with no mucus suspension. Coral Color Reference Card (Siebeck et al., 2006) was used as a standard reference to indicate healthy coral samples.
Experimental Design
In CT, samples were treated similarly to the condition during acclimatization. Meanwhile, the heat-stress experiment was assigned in ET by gradually increased the seawater temperature at 0.5∘C/12 h using an aquarium heater (SOBO aquarium heater 300W-HL) to obtain three temperature regimes (i.e., 27.0 ± 1.0, 29.0 ± 1.0, and 31 ± 1.0∘C). The highest temperature (31 ± 1.0∘C) was chosen following the natural bleaching event reported in Malaysia (Guest et al., 2012). The sample collection begins after 10 days of acclimatization by collecting the first sub-colonies in both tanks and represented as the samples on Day 1. Then, the seawater temperature in ET was gradually increased to 29.0 ± 1.0∘C (0.5∘C/12 h), and samples were collected after 24 h of exposure at 29.0 ± 1.0∘C and labeled as Day 3. A similar procedure was applied for Day 5 when the temperature reached 31 ± 1.0∘C.
The remaining samples were left for another 48 h of exposure at 31 ± 1.0∘C before the final collection (Day 7). Samples in CT were collected following the sampling frequency in ET (Day 1, Day 3, Day 5, and Day 7). The summary of the experimental design is illustrated in Figure 3. In total, 144 fragments were collected from both tanks and directly kept in a −80∘C freezer prior to the laboratory analysis. In the laboratory, the samples were divided accordingly for FA analysis, SD density, and enzymatic assays. For every sample collection (Day 1, Day 3, Day 5, and Day 7), three replicates of coral fragments were used for each of the analyses.
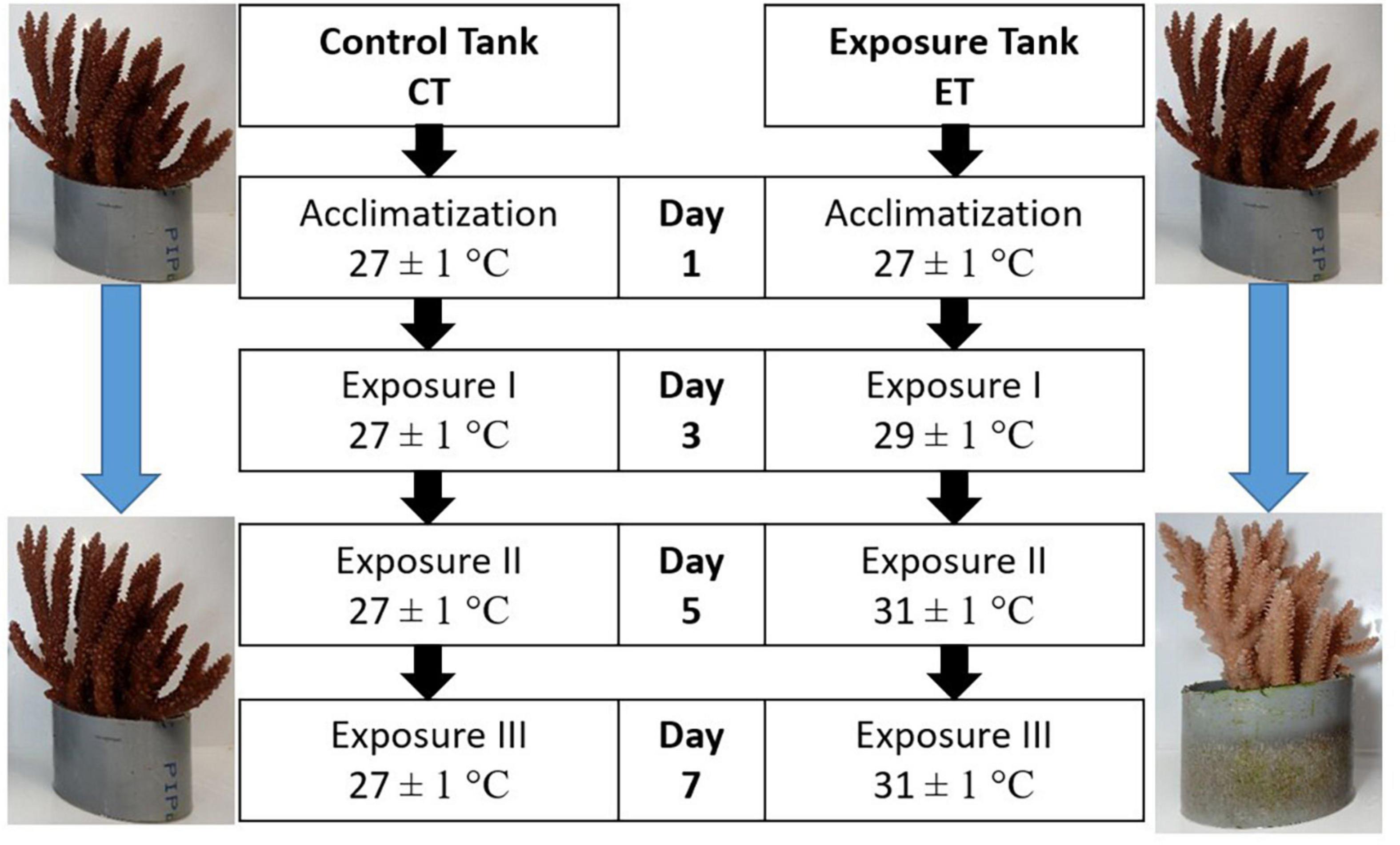
Figure 3. Experimental design for control tank (CT) and exposure tank (ET). Samples were collected after 10 days of acclimatization and regarded as Day1, Day 3, Day 5, and Day 7. Both tanks were sampled at the same time.
Preparation of Samples
Samples for FA analysis and SD density were prepared by removing the coral tissue from the skeleton using a jet of high-pressure air from an artist airbrush containing chilled distilled water (80 psi, approximately 1-cm distance from tip of airbrush to coral) into a thick, transparent polyethylene bag (Johannes and Wiebe, 1970). The tissue slurry was then poured into a 50-ml tube, and the plastic bag was rinsed using the airbrush to ensure full coverage of the remaining tissue on the plastic wall.
In contrast, samples for enzymatic assay were prepared by removing the coral tissue from the skeleton utilizing the jet of high-pressure air from an artist airbrush containing a phosphate-buffered saline solution. The slurry or homogenate that contained the tissue (host and symbiotic algae) was then transferred into 15-ml tubes and centrifuged (8,000 × g) at 4∘C for 15 min. The tissue-free supernatant was removed and replaced with 1 ml of homogenized buffer [0.01 M Tris–Cl, 0.5 M sucrose, 0.001 M ethylenediaminetetraacetic acid (EDTA), 0.15 M KCl, and pH 7.4]. The pellet was then homogenized using an ultrasonic cell disruptor (Misonix Microson XL2000) for 20-s sonication and 50-s rest for 10 cycles on ice. The homogenate was then centrifuged (18,000 × g) for 20 min at 4∘C. The coral protein extracts in the supernatant were harvested, the protein concentration was determined by the Bradford assay as previously described (Bradford, 1976), and the remaining coral protein extracts were transferred into a clean 1.5-ml tube and stored in a −80∘C freezer before further analysis.
Symbiodiniaceae Cell Count
The density of SD was determined using an hemocytometer (Neubauer-Improved Tiefe Depth Profondeur, 0.100 mm). The resultant slurry was shaken vigorously, and the sub-sample was dropped onto the hemocytometer and viewed under Leica DME compound microscope at 40 × magnification. The number of algae cells was calculated from the replicate of the hemocytometer count (n = 8). The SD density (×106 cell cm–2) was determined by dividing the total number of SD cells with a surface area of the denuded skeleton.
Fatty Acid Analysis
The remaining slurry was then filtered using a known weight of blank filter paper (Sartorius, Glass-Microfiber Disks, MGC, Ø 47 mm, and retention rate of 1.2 μm). Samples were freeze-dried (Labconco FreeZone 4.5-L Benchtop Freeze Dry System) for 12 h at −40∘C to remove the excess water and determine the dried coral tissue biomass. The dried samples were kept in the −20∘C freezer prior to FA analysis.
Samples of dried coral tissue were used for FA analysis using the One-Step method as outlined by Abdulkadir and Tsuchiya (2008). Samples amounting to 200–300 mg were mixed with 4 ml of hexane and 1 ml of internal standard solution in a 50-ml centrifuge glass tube. A glass rod was used to ensure that the sample was soaked with the mixture of hexane and internal standard solution. Of note, 2 ml of 14% BF3 in methanol and a magnetic stirrer bar were added to the tube. Rapidly, the headspace of the tube was flushed with nitrogen gas before the tube was tightly closed with a Teflon-lined screw cap. The tube was then transferred into a glass beaker of boiling water heated using a hot plate at 100∘C for 120 min under continuous stirring. Then, the tube was cooled at room temperature before adding 1 ml of hexane and 2 ml of distilled water. The tube was shaken vigorously and centrifuged at 2,500 rpm to obtain the fatty acid methyl ester (FAME) of the sample. Among the two layers formed, the upper layer was the hexane containing the FAME. Using a glass pipette, the layer of FAME was slowly transferred into a clean 5-ml sample vial, and the volume of the FAME collected was also recorded. All extracted FAMEs were then stored in a −20∘C freezer prior to the gas chromatography (GC) analysis.
The component and composition of FAMEs were identified using Shimadzu 2010 GC-Flame Ionization Detector (GC-FID), Shimadzu, Japan, equipped with acapillary column (Shimadzu Rtx-2330 30 m × 0.25 mm internal diameter × 0.25 μm film thickness) and connected with a computer possessed of GC Solution (Shimadzu,Japan) software, autosampler, and splitless injection system, and connected with computer possessed GC Solution software. The injection volume was 1 μl, and the injector and detector temperatures were 300 and 270∘C, respectively. The temperature program was set at 60∘C held for 2 min, then from 60 to 160∘C at 20 min, and held at 240∘C for 10 min. Helium was used as a carrier gas and stream at a constant flow of 1.5 ml min–1. Each component of FAMEs identified was compared with known external standards (FAME-37 Sigma–Aldrich, St. Louis, MO, United States, bacterial acid methyl esters (BAME), and Cod Liver Oil). The resulting peak was used to determine the FA composition.
Enzymatic Analysis
All of the chemicals used in the enzymatic analysis were obtained from Fisher Scientific, Loughborough, United Kingdom (Tris Base, Tris–Cl, sucrose, potassium dihydrogen phosphate, and KCl), Merck, Germany (NaOH, H2O2, and dipotassium hydrogen phosphate), Sigma–Aldrich, St. Louis, MO, United States [EDTA, 1-chloro-2,4-dinitrobenzene (CDNB), and reduced glutathione (GSH)], R&M Chemicals, United Kingdom (paraffin wax), and HmbG Chemicals, Hamburg, Germany (ethanol).
The enzymatic activity of catalase (CAT) was assessed by using a spectrophotometric method to measure the breakdown of H2O2 by CAT enzyme by following the study of Beers and Sizer (1952). Coral protein extracts were diluted with phosphate buffer (50 mM, pH 7.0) in a quartz cuvette and incubated to reach room temperature (±25∘C). Immediately, 59 mM H2O2 was added while blank was set with a similar condition without the presence of protein sample extracts. The decrease in absorbance was measured for 120 s by using UV-spectrometer (Shimadzu UV-1800 UV–Vis Spectrophotometer), and the enzyme activity was calculated based on the extinction coefficient of H2O2 at Δ240 nm (43.6 M–1 cm–1). The unit of CAT activity is defined as decomposition of 1 μM H2O2 to O2 and water per minute at pH 7.0 at 25∘C and final substrate absorbance at 240-nm decay at a constant rate of 0.3–0.5.
The enzymatic assay for glutathione S-transferase (GST) activity was adapted from the study by Habig and Jakoby (1981) using the CDNB and GSH as substrates. Coral protein extracts were diluted with phosphate buffer (150 mM, pH 6.5) and 20 mM CDNB in a 96-well plate. The reaction was started by adding GSH, and immediately, the enzyme activity was determined spectrophotometrically at 340 nm using a microplate reader (Molecular Devices, SpectraMax® iD3 Multi-Mode Microplate Reader) at every 30 s for 5 min. The GST activity was calculated based on the absorbance increase per minute, using the CDNB extinction coefficient (340 nm = 9.6 mM–1 cm–1). The unit of GST activity is defined as enzyme-conjugated 10 nmol of CDNB with the presence of GSH per minute in 1 mg of protein.
Superoxide dismutase activity was measured using the SOD Determination Kit (Sigma–Aldrich, St. Louis, MO, United States) following the instructions of the manufacturer. In brief, the reduction of oxygen to superoxide anion is detected by tetrazolium salt and produces formazan dye, which can be detected spectrophotometrically. In a 96-well plate containing coral protein extracts with and without superoxide-producing enzymes, absorbance at 450 nm was measured, compared, and standardized by the weight of protein used. Results were expressed in U per mg protein, where U is defined as the amount of enzyme necessary to produce 50% inhibition of the reduction rate of tetrazolium salt measured at 450 nm.
Statistical Analysis
Prior to statistical analysis, the normality of data sets comprised of SD density, antioxidant activities (CAT, GST, and SOD), and FA composition (FA classes, FA components, and putative FA health indicator) was tested by using the Shapiro–Wilk test and homogeneity test. However, most of the data sets were not normally distributed even after the arsine transformed; hence, the Kruskal–Wallis one-way ANOVA on ranks was used. The data were tested for differences among the samples collected from each tank (n = 9 per collection). Any data with a p-value less than 0.05 were considered significant and further analyzed using a pairwise multiple comparison test. To seek the relationship of SD densities with associated variables (CAT, GST, SOD, and FA composition), the Spearman’s correlation was performed to the ET data sets to understand the changes during the experiment. All analyses were performed using the statistical software SPSS IBM.
Results
Symbiodiniaceae Density
Symbiodiniaceae density in CT and ET during the experimental period changed over the experimental period (Figure 4). In CT, acclimatization temperature did not significantly affect the SD density (Kruskal–Wallis H = 4.345, p = 0.227). Daily observation showed no physical evidence of stress (polyp retraction, paleness or bleaching, and/or tissue rupture) among the samples. In contrast, samples in ET displayed a significant decline in mean SD densities (Kruskal–Wallis H = 25.887, p < 0.001) especially at 31 ± 1.0∘C during Day 5 (1.19 ± 0.15 × 106 cells cm–2) and continued to drop (0.71 ± 0.06 × 106 cells cm–2) until the end of experiment. Even though the loss of SD densities occurred at Day 5, no physical evidence of stress (polyp retraction, paleness or bleaching, and/or tissue rupture) among the samples was observed within the period. No significant change was found during temperature changes at 27 ± 1.0 and 29 ± 1.0∘C (Day 1 vs. Day 3, p = 0.248, adjusted significance = 1.000).
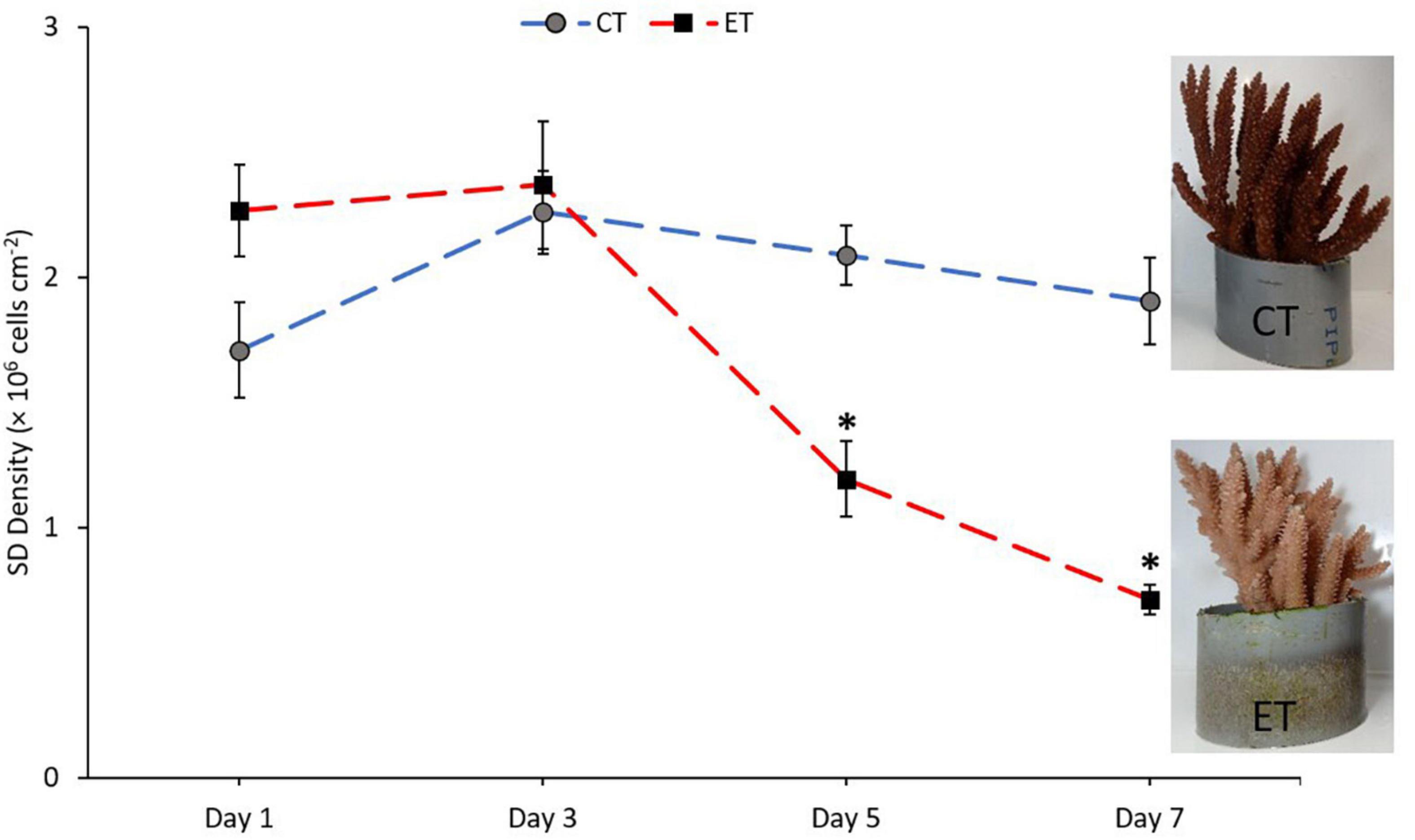
Figure 4. Mean densities of Symbiodiniaceae in CT and ET throughout the experimental period with the images of samples taken from ET and CT during the end of experiment. All data points are mean ± SE (n = 9). Asterisk represent significant difference (p < 0.05).
Antioxidant Enzyme Activities
In this study, antioxidant enzyme activities of CAT, GST, and SOD in the samples from both tanks were measured and shown in Figure 5. The activity of CAT in ET (Kruskal–Wallis H = 14.651, p = 0.002) and CT (Kruskal–Wallis H = 13.116, p = 0.04) was significantly different among the samples during the experimental period (Figure 5A). In both tanks, the activity of CAT increased significantly during Day 5 (p < 0.05) as revealed using the pairwise test. Even though the pattern of CAT activity in both tanks was relatively similar, the CAT activity in ET continued to decrease significantly as compared with CT during the end of experiment (Day 5 vs. Day 7; ET, p = 0.001, adjusted significance = 0.003, CT; p = 0.235, adjusted significance = 1.000).
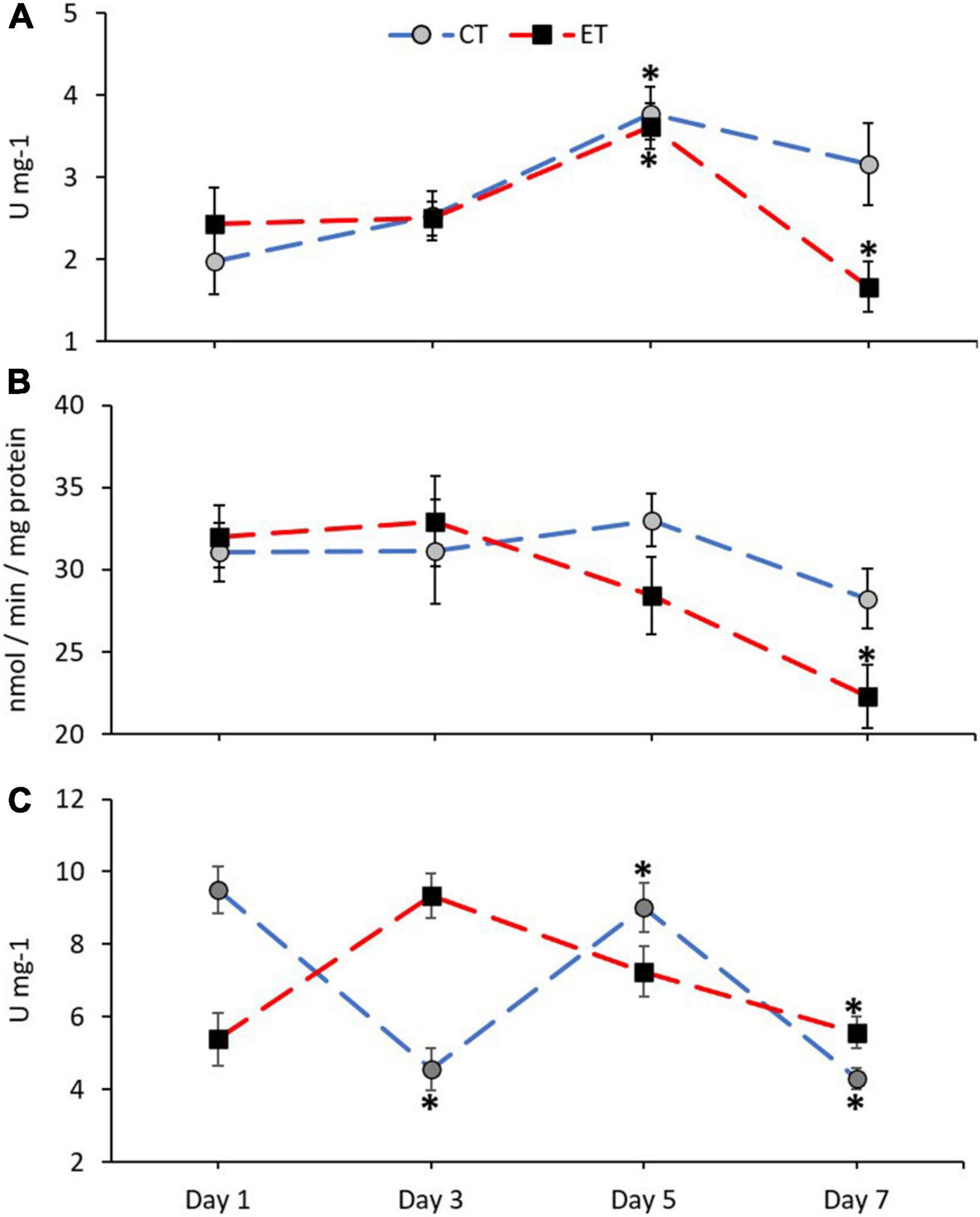
Figure 5. Enzymatic activity of catalase, CAT (A), glutathione S-transferases, GST (B), and superoxide dismutase, SOD (C) in coral samples from CT and ET during the experimental period. All data points are mean ± SE (n = 9). Asterisk represents a significant difference (p < 0.05).
No clear changes of GST activity were found in CT (Kruskal–Wallis H = 3.068, p = 0.381) as compared with ET (Kruskal–Wallis H = 10.325, p = 0.016; Figure 5B). In comparison with the samples in ET, samples taken during the end of experiment (Day 7) contained significantly lower GST activity than the samples from Day 1 (p = 0.006, adjusted significance = 0.038) and Day 3 (p = 0.005, adjusted significance = 0.029).
The activity of SOD in ET (Kruskal–Wallis H = 16.924, p = 0.001) and CT (Kruskal–Wallis H = 23.603, p < 0.001) was significantly different among the samples during the experimental period (Figure 5C). However, in ET, a gradual decrease of SOD activity can be observed on Day 7 as compared with Day 3 (Day 3 vs. Day 7; ET, p = 0.001, adjusted significance = 0.003).
Fatty Acid Composition
Composition of SAFA, MUFA, and PUFA
The mean composition of SAFA (Kruskal–Wallis H = 10.900, p = 0.012), monounsaturated fatty acid (MUFA; Kruskal–Wallis H = 10.063, p = 0.018), and PUFA (Kruskal–Wallis H = 8.187, p = 0.042) was significantly changed between the samples in ET (Figure 6). In both tanks, the proportion of SAFA, MUFA, and PUFA remained stable from Day 1 to Day 5. In CT, PUFA composition significantly increased (Day 5 vs. Day 7; p = 0.008, adjusted significance = 0.047) during the end of experiment. Meanwhile, MUFA (p = 0.003, adjusted significance = 0.015) and PUFA (p = 0.007, adjusted significance = 0.044) drastically decreased, while SAFA composition increased significantly (p = 0.003, adjusted significance = 0.020) when the samples exposed to temperature of 31∘C.
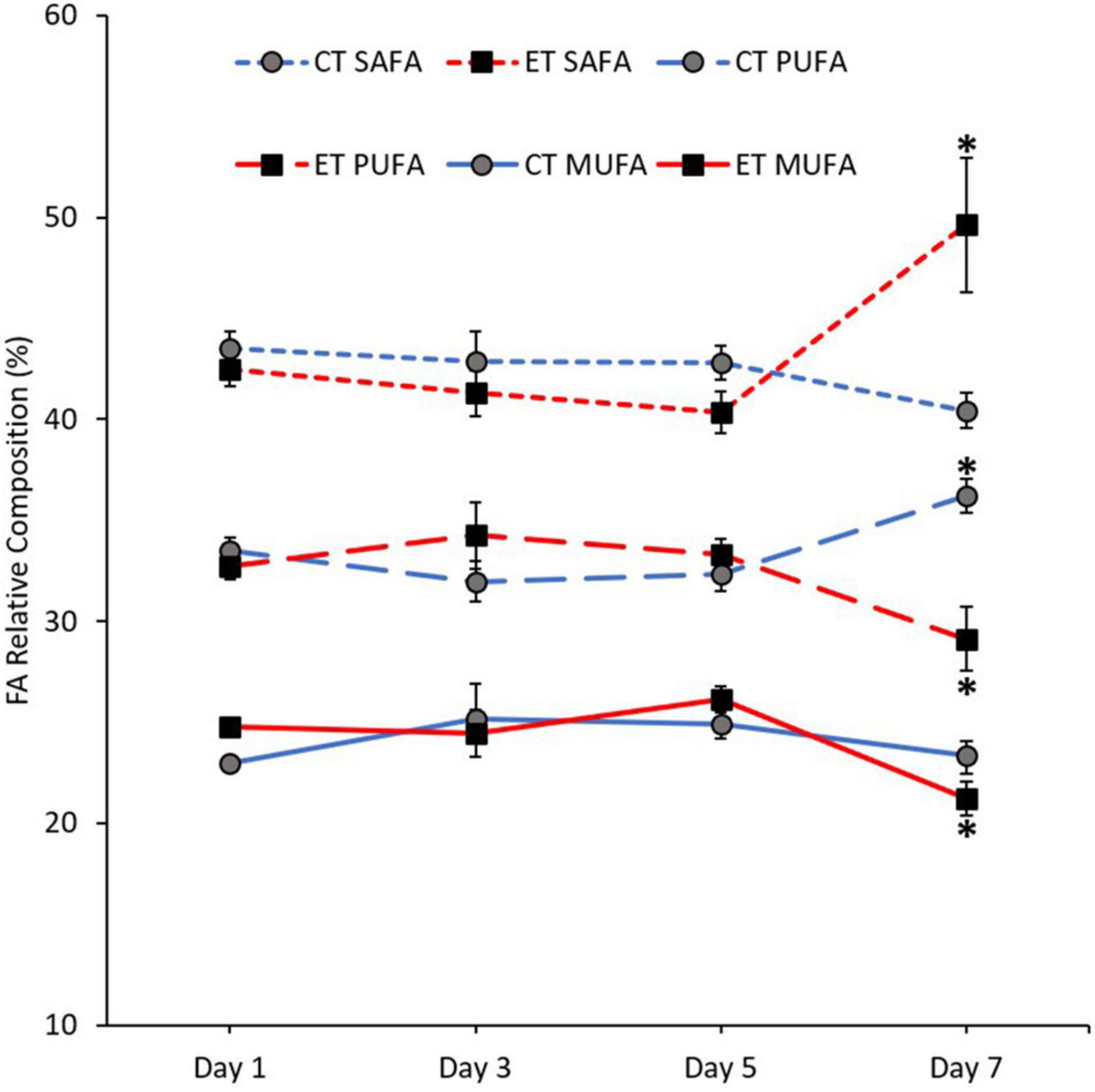
Figure 6. Composition of fatty acid (FA) classes [saturated fatty acid (SAFA), monounsaturated fatty acid (MUFA), and polyunsaturated fatty acid (PUFA)] in coral samples from CT and ET during the experimental period. All data points are mean ± SE (n = 9). Asterisk represents a significant difference (p < 0.05).
Polyunsaturated Fatty Acid Markers
In this study, the pattern of increase and decrease of FA markers (18:3n6, 18:4n3, 20:5n3, and 22:6n3) was observed in both tanks. Except for 18:4n3 (CT; Kruskal–Wallis H = 4.187, p = 0.242, ET; Kruskal–Wallis H = 7.006, p = 0.072; Figure 7B), other markers show a significant change of FA composition during the experimental period. As shown in Figure 7A, no significant change of 18:3n6 composition was found in CT (Kruskal–Wallis H = 4.373, p = 0.224) but the composition decreased significantly in ET (Kruskal–Wallis H = 18.584, p < 0.0.01) especially when the samples were exposed from 29 ± 1.0 to 31 ± 1.0∘C (p < 0.001, adjusted significance = 0.002). Meanwhile, the composition of 20:5n3 in CT increased significantly (Kruskal–Wallis H = 12.111, i = 0.007) from Day 3 to Day 7 (p = 0.003, adjusted significance = 0.019; Figure 7C). In contrast, the marker composition was significantly changed in ET (Kruskal–Wallis H = 19.649, p < 0.001), whereas the composition was decreased significantly during the end of experiment (Day 7 vs. Day 1, 3, and 5; p < 0.05, adjusted significance < 0.05). Slightly lower 22:6n3 composition in ET (Kruskal–Wallis H = 5.056, p = 0.168) was relatively observed as compared with CT (Kruskal–Wallis H = 12.842, p = 0.005), which showed a significant increase in the composition of these markers (Figure 7D).
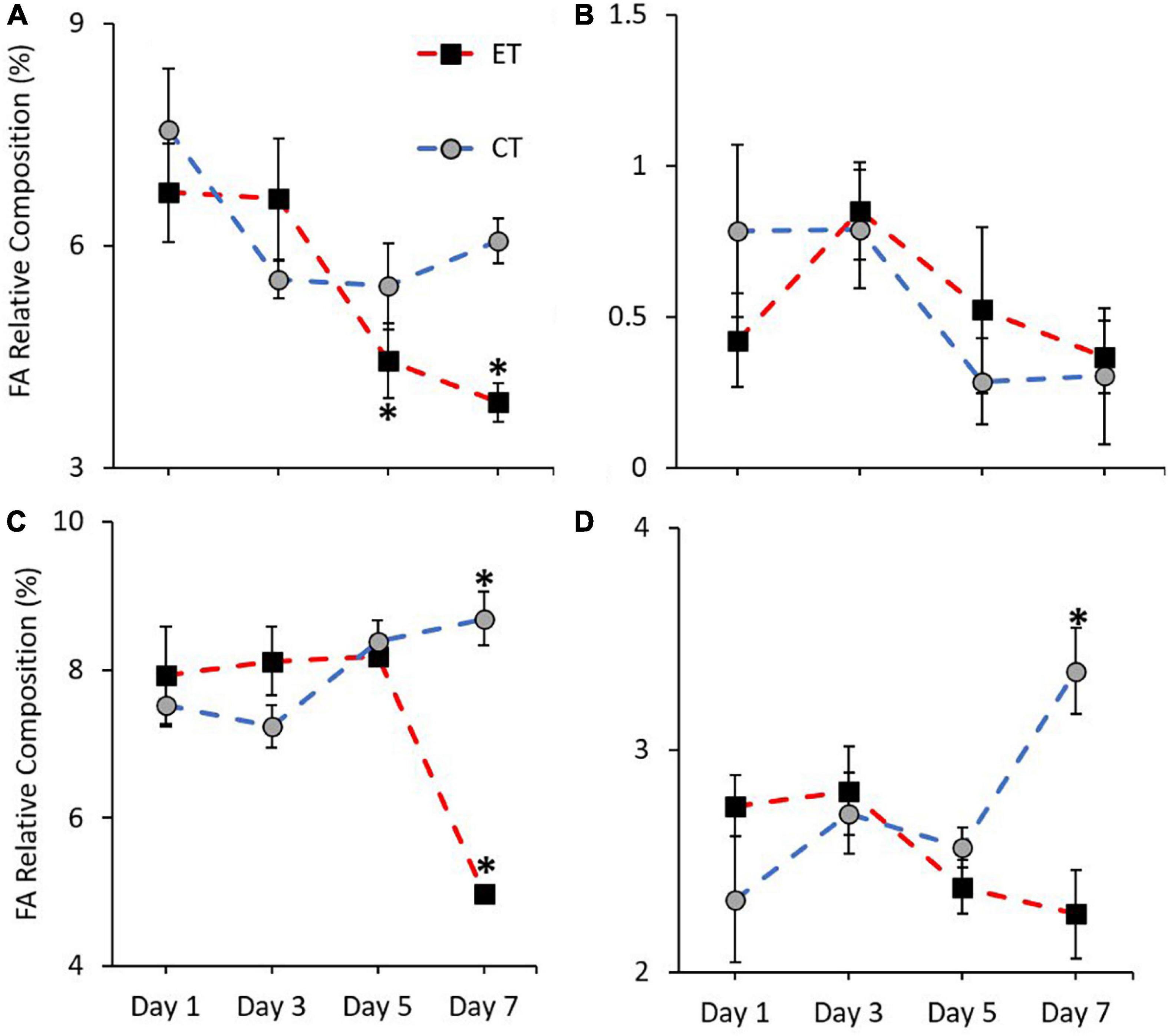
Figure 7. Composition of most affected PUFA markers (A = 18:3n6, B = 18:4n3, C = 20:5n3, and D = 22:6n3) in coral samples from CT and ET during experimental period. All data points are mean ± SE (n = 9). Asterisk represents a significant difference (p < 0.05).
Putative Fatty Acid Health Indicator
Figure 8 shows the ratio of FA health indicators examined in CT and ET. Samples in both tanks show a clear difference in n-3 LC:n-6 LC (CT; Kruskal–Wallis H = 14.892, p = 0.002, ET; Kruskal–Wallis H = 12.766, p = 0.005) and DHA:ARA (CT; Kruskal–Wallis H = 9.855, p = 0.020, ET; Kruskal–Wallis H = 16.770, p = 0.001) and also show relatively similar trend of increase and decrease (Figures 8A,C). However, the ratios were significantly lowered in ET as compared with CT, especially during the samples exposed to higher temperature from 29 ± 1.0 to 31 ± 1.0∘C. Conversely, no clear difference was observed for EPA:ARA in CT (Kruskal–Wallis H = 2.471, p = 0.480) as compared with ET (Kruskal–Wallis H = 18.213, p < 0.001). In ET, the EPA:ARA drastically decreased when the samples were treated at 31 ± 1.0°C (Figure 8B).
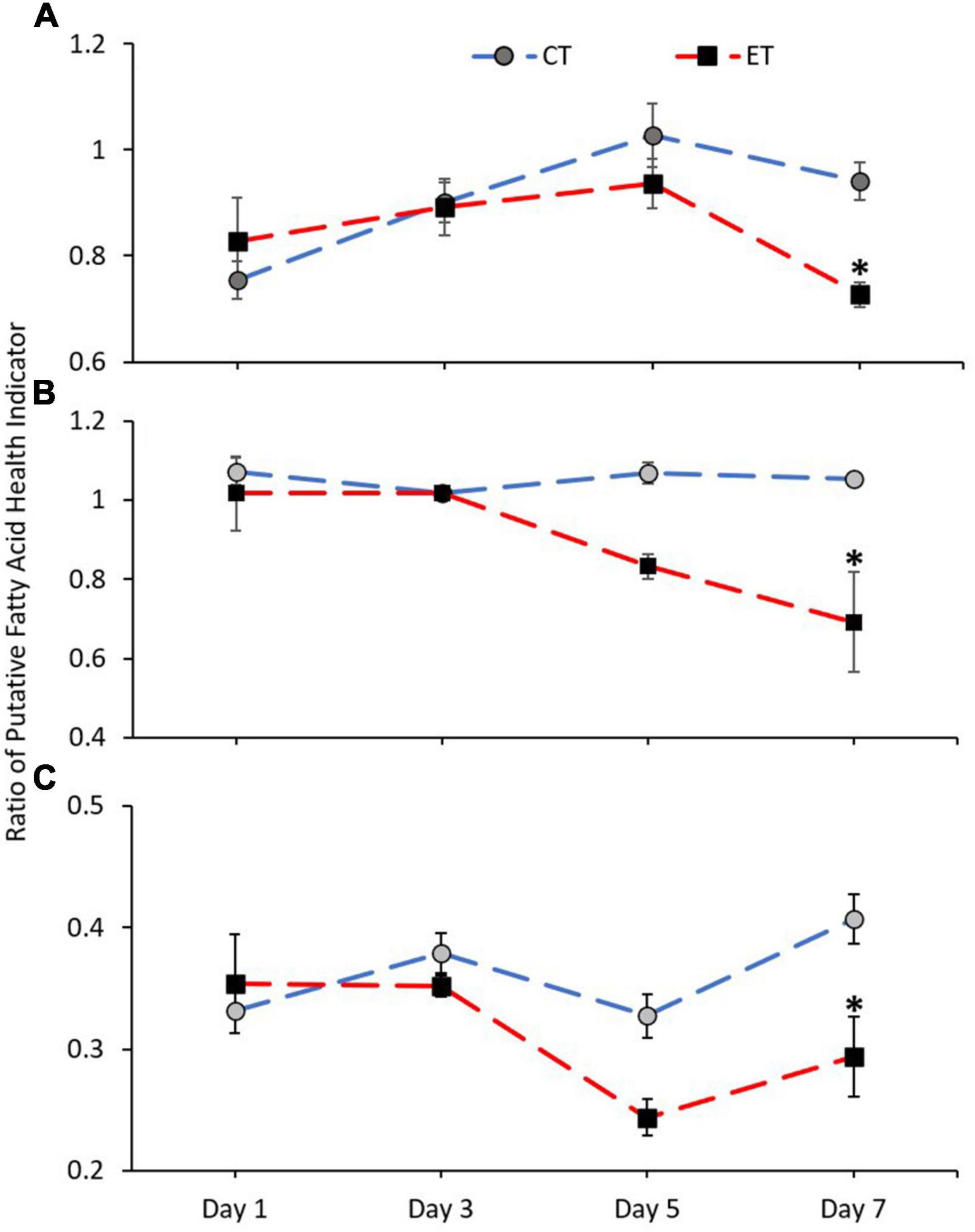
Figure 8. Ratio of coral health indicators (A = n-3 LC:n-6 LC, B = EPA:ARA, and C = DHA:ARA) in coral samples from CT and ET during the experimental period. All data points are mean ± SE (n = 9). Asterisk represents a significant difference (p < 0.05).
Correlation With Symbiodiniaceae Density
In this study, a positive correlation was found between the enzymatic activity, FA markers, and health indicator ratio with the SD densities (Table 1). As the temperature increased, the SD density was positively correlated with GST activity, 18:3n6, 20:5n3, 22:6n3, and the ratio of n-3 LC:n-6 LC, EPA:ARA, and DHA:ARA.
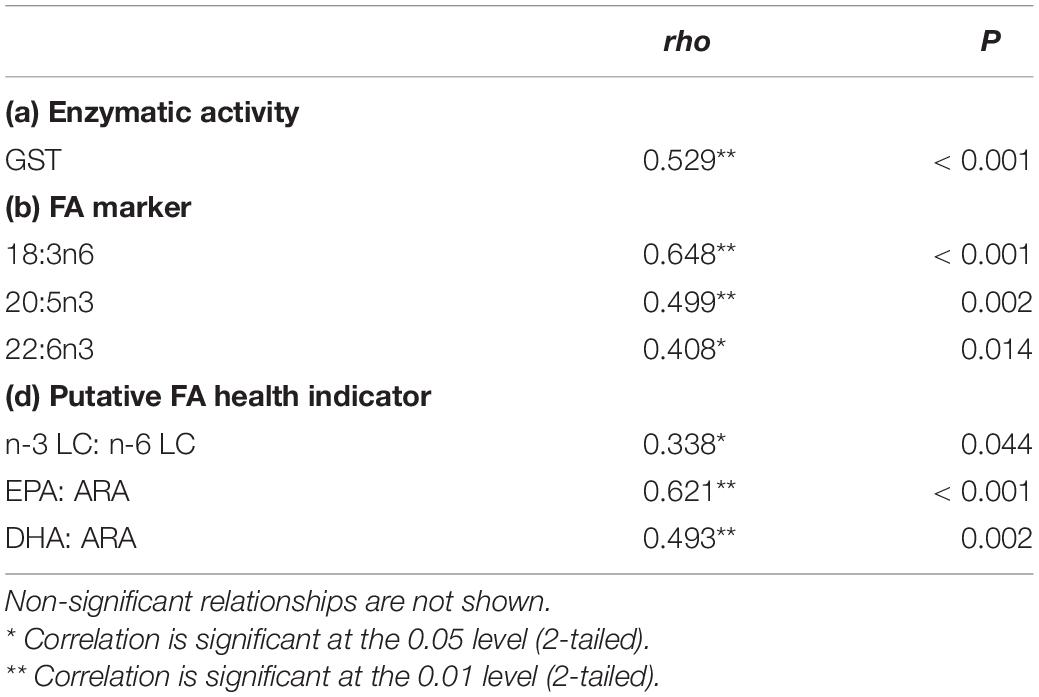
Table 1. Summary of significant correlation between SD density with enzymatic activity, fatty acid marker, and putative FA health indicator from data obtained in experimental tank data.
Discussion
Effect of Thermally Induced Stress
This study revealed the effect of heat stress on SD density, enzymatic activities, and FA composition in shallow-water coral, A. digitifera, via an experimental approach. The results clearly show that the bleaching response was obvious when the samples were exposed to 31 ± 1.0∘C, which caused paleness or bleaching as a result of a significant loss of SD density from the corals. The symptom of bleaching was best explained by the expulsion of symbiotic algae from their host (Fitt et al., 2000; Imbs and Yakovleva, 2012; Rodríguez-Troncoso et al., 2016). In a prolonged heat exposure, the rate of symbiotic algae release is higher with the degraded forms of symbiotic algae among the expelled population increased in number. Fujise et al. (2014) also found that the proportion of degraded cells in hospice was low, whereas it was high in expelled populations. Therefore, heat stress can cause deterioration to the symbiotic algae, and the removal of symbiotic algae by the coral is an important mechanism to counterbalance the overproduction of ROS by symbiotic algae (Downs et al., 2002; Cunning and Baker, 2012).
Oxidative stress induced by elevated seawater temperature affected both corals and their symbiont that causes cellular damage, and the expulsion of the algae is due to the elimination or accumulation of toxic ROS (Lesser, 2006, Lesser, 2011; Baird et al., 2008; Weis, 2008). In normal conditions, the formation of radicals and the antioxidant system are well regulated to maintain the steady-state concentration of ROS at low levels involving antioxidant enzymes such as SOD, CAT, ascorbate peroxidase, GST, and glutathione peroxidase (Lesser, 2006; Rewitz et al., 2006; Lushchak, 2011). This can be observed in CT where our representative CAT, GST, and SOD antioxidant activities were normally high or well regulated during the steady-state of SD density as compared with the coral with reduced algae densities in ET. After 4 days of exposure at 31 ± 1.0∘C, A. digitifera cannot recover their antioxidant defenses even the SD density has been at the lowest. During this condition, antioxidant enzyme activities can counterbalance with other oxidative damage products, but species that withstood longer thermal exposure could present low levels of antioxidant enzyme activities due to the exhaustion of enzyme production (Yakovleva et al., 2004; Dias et al., 2019). This is shown by the gradual decrease of enzymatic activity (GST and CAT) as demonstrated in this study. CAT activity in ET significantly decreases once corals lose their symbiont (Day 7) as compared with CT, indicating that the accumulation of H2O2 was significantly decreased. According to the theory of oxidative stress of coral bleaching, H2O2 generated by symbiotic algae can diffuse into coral cytoplasm, hence jeopardizing the antioxidant defenses of coral (Downs et al., 2002; Lesser, 2006). Prolonged exposure to high temperature can also damage ROS-sensitive enzymes, as observed by the decrease of CAT, GST, and SOD at the end of the experiment (Yakovleva et al., 2004; Dias et al., 2019). Therefore, we suggested that at high SD density, corals are vulnerable to oxidative stress, but prolonged exposure to heat can damage the enzymatic network and can be overwhelmed by ROS production. This is consistent with other studies that observed corals with high SD density are more susceptible to bleaching (Cunning and Baker, 2012; Xu et al., 2017) as the symbiotic algae produce harmful substances under high temperature stress, which cause tissue damage to their host (Nesa and Hidaka, 2009; Yakovleva et al., 2009). Therefore, it is suggested that the overproduction of harmful ROS induced by prolonged heat stress regulate the SD density and antioxidant activities in A. digitifera.
During stress condition, corals significantly rely on their energy reserve, namely, FAs. The trend of increased SAFA and decreased PUFA composition during coral bleaching under heat stress has been well documented (Bachok et al., 2006; Imbs and Yakovleva, 2012). The reduction of unsaturated FAs during stress condition is known to be related to lipid peroxidation (Okuyama et al., 2008; Ayala et al., 2014). Lipid peroxidation occurred when the primary defense by the antioxidant system overwhelms, causing oxidative damage to the lipid (Baird et al., 2008). During lipid peroxidation, the ROS attack lipid containing carbon–carbon double bond(s) such as MUFA and PUFA (Okuyama et al., 2008; Parrish, 2013; Ayala et al., 2014) because the double bond in unsaturated FA weakens the C–H bond on the carbon atom nearby to the double bond, which makes it more susceptible to oxidative stress (Catalá, 2010). The cell membrane consists of lipid that contains PUFA that is highly susceptible to toxic ROS. The hydrophobic characteristic of PUFA might prevent the entry of hydrophilic H2O2 molecules (Okuyama et al., 2008). Hence, PUFA composition can be the response of a coral to maintain membrane integrity and function during stress (Papina et al., 2007). At present, lower enzymatic activities during bleaching show that the antioxidant system is unable to tolerate the overproduction of toxic ROS, leading to oxidative damage that resulted in lower MUFA and PUFA composition. A significant decrease of FA composition in both classes during the thermally induced experiment shows that corals utilized their unsaturated FA as a final barrier or as a repair system toward oxidative damage (Baird et al., 2008). The membrane-shielding effect of PUFA, especially the long-chain n-3 series are likely to function as a primary protective barrier against the ROS (Okuyama et al., 2008). MUFA composition significantly decreased during the experiment. In contrast, the high composition of MUFA in bleached corals was observed due to the increase in bacteria colonization, which is proven by the presence of bacterial markers such as 16:1n7 and 18:1n7 (Bachok et al., 2006). However, in an experimentally control environment, the composition of these markers was found in trace amount. Meanwhile, the trend of increased SAFA in bleached corals is also related to the aforementioned lipid peroxidation (Parrish, 2013; Ayala et al., 2014) as well as to increase the rigidity of cellular membranes (Papina et al., 2007). Moreover, SAFA is the least reactive chemical and more stable than unsaturated FAs (Ratnayake and Galli, 2009).
Fatty Acid Markers
One of the particular interests in this study was the reduction in certain PUFA compositions such as 18:3n6, 18:4n3, EPA (20:5n3), and DHA (22:6n3). In this experiment, heat stress induced the reduction of 18:3n6 and 18:4n3 composition, which was similarly observed in other thermally induced experiments toward A. intermedia and Montipora sp. (Imbs and Yakovleva, 2012). Meanwhile, bleached corals such as Pavona sp., Acropora sp., and Goniastrea sp. contained significantly lower 18:4n3 and 22:6n3 than healthy corals, indicating that the loss of symbiotic algae from the host has influenced the FA composition (Bachok et al., 2006). The 18:3n6, 18:4n3, 20:5n3, and 22:6n3 were recognized as a possible FA characteristic in diatoms and dinoflagellates as well as recognized as symbiotic algae markers in hard and soft coral (Papina et al., 2003; Imbs and Dautova, 2008; Imbs et al., 2010b,c, 2014). It is suggested that the presence of specific markers to the symbiotic algae in the host tissue indicates that there is a translocation of specific PUFA markers from symbiotic algae to the host (Papina et al., 2003; Imbs et al., 2010b). Therefore, changes in SD densities during the experiment should be correlated with the FA markers. Among the PUFAs, the 18:3n6, 20:5n3, and 22:6n3 were positively correlated with the SD density, hence, a possible marker for symbiotic algae. No significant correlation was found in 18:4n3, and the composition was not significantly changed in both CT and ET, showing that the FA possibly represents the host marker. Several PUFAs can be synthesized by the host coral tissue via de novo synthesis (Monroig et al., 2013) and marked as the host markers (Treignier et al., 2008; Imbs et al., 2010a, b). To be clear, throughout the experimental period, the corals were not fed; thus, the effect of heterotrophy feeding is not significant, and this leaves the coral to rely on autotrophy for their daily energy requirement. Therefore, as the heterotrophy effect is limited, the 18:4n3 can be considered as the host marker.
Polyunsaturated fatty acids such as EPA and DHA are known to be involved in anti-inflammatory processes. PUFA from n-6 series such as 20:4n6 (ARA) is required for the synthesis of pro-inflammatory health hormones (Calder, 2017; Kumar et al., 2019). Inflammation is known as a defense mechanism that protects the host from stress (Calder, 2010). As shown in Figures 7C,D, the significant loss of EPA, DHA, and SD density in ET was observed after exposing the coral to the higher temperature. As the SD density is reduced, the coral loses the translocation of EPA and DHA from symbiotic algae, hence, increasing the possibility of excessive inflammation on the coral tissue under a prolonged bleaching condition (Innes and Calder, 2018).
Fatty Acid Health Indicator
Healthy corals can be indicated by a higher ratio of PUFA n-3 LC:n-6 LC (Dalsgaard et al., 2003). By the end of the experiment, all three PUFA ratios in the coral tissue of A. digitifera exposed to heat stress were significantly lower than the control condition. This is consistent with the field data showing that healthy corals contained a higher ratio of the PUFA n-3 LC:n-6 LC as compared with unhealthy corals (Rocker et al., 2019; Kim et al., 2021). Significant loss of the n-3 LC:n-6 LC in ET at the end of the experiment coincides with the significant loss of SD density, indicating some of these PUFAs, which were heavily transported to coral by their symbiotic algae, have been reduced. This is further proved by a positive correlation between the n-3 LC:n-6 LC with the SD density, showing that the n-3 LC PUFA is mainly obtained from the symbiotic algae. Under certain circumstances, n-3 LC:n-6 LC ratio may be preserved via heterotrophy and autotrophy feeding (Rocker et al., 2019). It is suggested that corals tend to modulate their feeding modes under certain conditions to provide an adaptive mechanism for sustaining their growth under stressful conditions (Grottoli et al., 2004; Teece et al., 2011). When the autotrophy mode is limited, corals sustain their metabolism by heterotrophy feeding (Treignier et al., 2008). For instance, bleached Montipora capitata extensively obtained their daily energy requirement via heterotrophic mode to assimilate lipid classes from zooplankton-derived carbon (Rodrigues et al., 2008). However, in our case, the corals in both tanks were not fed throughout the experiment; hence, the mechanism of switching the feeding mechanisms can be negligible. Naturally, the balance among this ratio is influenced by mixotrophic mechanisms, via autotrophy and heterotrophy feeding. Therefore, in this case, the balance is interrupted by limiting the heterotrophy feeding, and the corals extensively rely on their autotrophy mechanism.
The EPA and DHA were known as the FA characteristics for symbiotic algae and cannot be synthesized by the animals (Figure 9). At present, when heterotrophy feeding is reduced, coral heavily relies on symbiotic algae to obtain the EPA and DHA as shown in Figure 9. This is further proven by the positive correlation between EPA:ARA and DHA:ARA with the SD density. EPA and DHA are markers for symbiotic algae while ARA was suggested to be synthesized by the host (Treignier et al., 2008; Imbs et al., 2010a, b). Reduction in both ratios shows the loss of SD density affecting the EPA and DHA composition while the host preserved the ARA composition. The ARA can be synthesized by the host through the joint action of desaturase and elongase enzymes as follows: 18:3n6 → 20:3n6 → 20:4n6 (Figure 9). This is coincided with the decrement of 18:3n6 composition (Figure 7A), possibly indicating that the host maintained their ARA composition by synthesizing other PUFAs. The high composition of ARA is also related to heterotrophy feeding (Rocker et al., 2019), but limited heterotrophy feeding had required the corals to manipulate other FA to continue synthesizing the ARA.
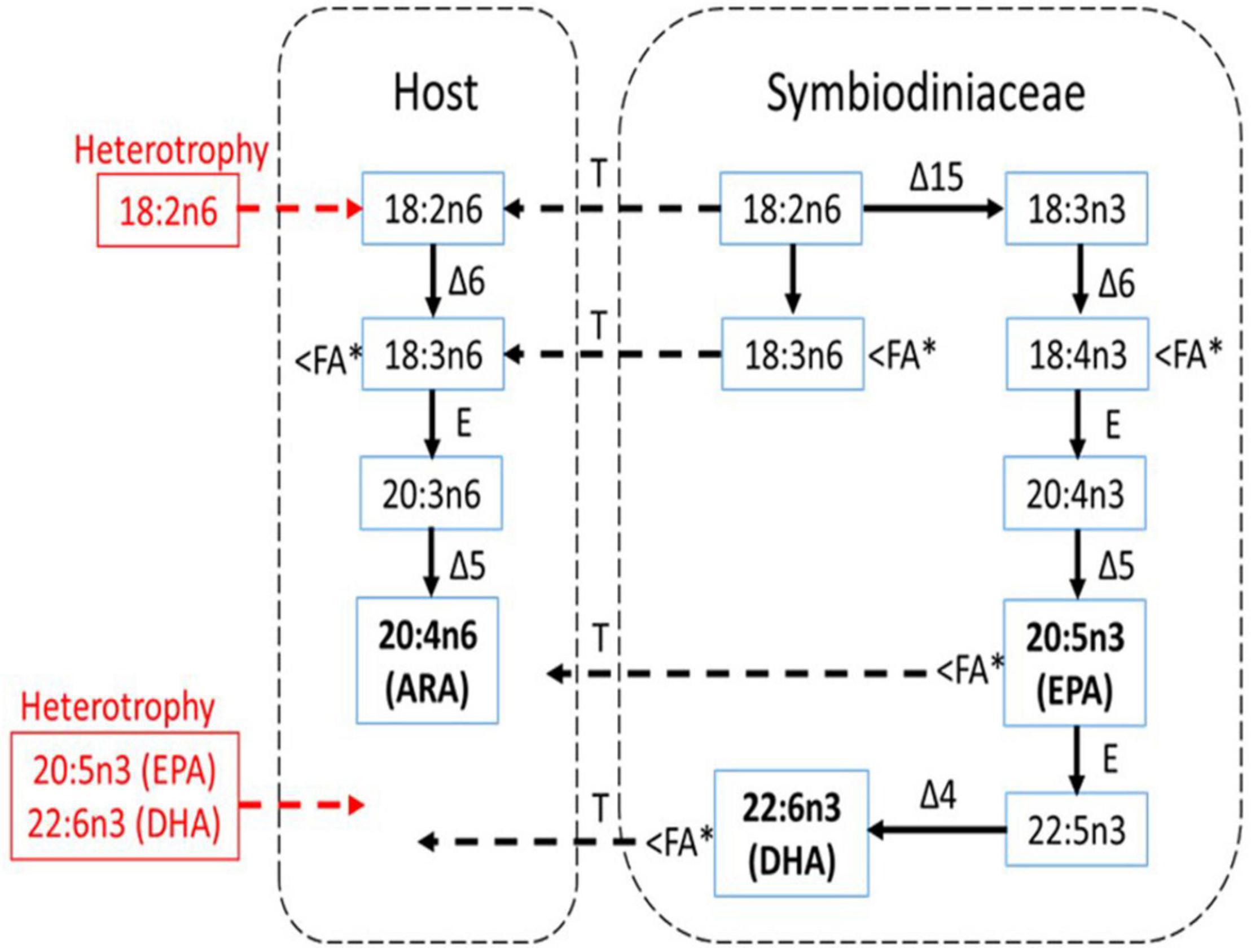
Figure 9. The pathway of biosynthesis of n-3 and n-6 PUFA in shallow-water coral (A. digitifera) and a scheme for the possible transport of these FA between the host and symbiotic algae (Symbiodiniaceae) under experimental condition (modified from Imbs et al., 2010b). Essential FA components (ARA, EPA, and DHA) are bold while the red color shows the essential FAs that are not translocated from heterotrophy feeding. Δ4, Δ5, Δ6, and Δ15 are corresponding desaturase; E, elongase; T, transport. The < FA∗ indicates FA component that was significantly reduced during the thermally induced experiment.
Conclusion
At present, the heat stress has induced coral bleaching on shallow-water hard coral, A. digitifera, which causes significant changes in SD density, antioxidant enzyme activities, FA composition, and putative FA health indicator. Prolonged stress had caused the coral to expel their symbiont to counterbalance the stress condition. However, the significant decrease of CAT, GST, and SOD indicates that the antioxidant enzyme activities were overwhelmed by the overproduction of harmful ROS, and coral was unable to cope with the endogenous stress. As the first barrier against the stress collapsed, coral expels their symbiotic algae and regulates their energy reserved via FA to restore healthy homeostasis. A significant decrease in unsaturated FA (MUFA and PUFA) significantly correlated with the loss of SD density and was associated with their susceptibility to harmful ROS. An increase in SAFA composition shows that the FA remains stable against the stress condition as compared with unsaturated FA. Additionally, a significant decrease in certain PUFA markers (18:3n6, 20:5n3, and 22:6n3) and putative FA health indicators (n-3 LC:n-6 LC, EPA:ARA, and DHA:ARA) was associated with the loss of SD density. Perhaps, limited heterotrophy feeding during the experimental period makes the coral highly dependent on their symbiotic partners.
Data Availability Statement
Written informed consent was obtained from the relevant individual(s) for the publication of any potentially identifiable images or data included in this article.
Author Contributions
CS: conceptualization, methodology, formal analysis, investigation, writing—original draft, writing—review and editing, and visualization. MS: conceptualization, methodology, investigation, and writing—review and editing. SJ: writing—review and editing, supervision, and project administration. CT: writing—review and editing and supervision. ZB: writing—review and editing, supervision, project administration, and funding acquisition. All authors contributed to the article and approved the submitted version.
Funding
This study was supported by the Ministry of Higher Education Malaysia, Fundamental Research Grant (FRGS; Vot No. 59538), Collaborative grant IPC (International Petroleum Corp.; Vot No. 53392), and Higher Institution Center of Excellence (HICoE), the Institute of Oceanography and Environment (Vot No. 66928).
Conflict of Interest
The authors declare that the research was conducted in the absence of any commercial or financial relationships that could be construed as a potential conflict of interest.
Publisher’s Note
All claims expressed in this article are solely those of the authors and do not necessarily represent those of their affiliated organizations, or those of the publisher, the editors and the reviewers. Any product that may be evaluated in this article, or claim that may be made by its manufacturer, is not guaranteed or endorsed by the publisher.
Acknowledgments
The authors would like to express their gratitude to all the researchers and staff for their invaluable assistance during the study period especially Puteri Nurshazmimi Zaidi for helping us in the hatchery. All types of equipment were provided by the Institute of Oceanography and Environment. The experiment was conducted in the hatchery of the Institute of Tropical Aquaculture and Fisheries.
Abbreviations
SD, Symbiodiniaceae; MUFA, monounsaturated fatty acid; PUFA, polyunsaturated fatty acid; LC, linolenic acid; EPA, eicosapentaenoic acid; ARA, arachidonic acid; DHA, docosahexaenoic acid; SAFA, saturated fatty acid; CT, control tank; ET, exposure tank; ROS, reactive oxygen species; FA, fatty acid.
References
Abdulkadir, S., and Tsuchiya, M. (2008). One-step method for quantitative and qualitative analysis of fatty acids in marine animal samples. J. Exp. Mar. Biol. Ecol. 354, 1–8. doi: 10.1016/j.jembe.2007.08.024
Apprill, A. (2020). The Role of Symbioses in the Adaptation and Stress Responses of Marine Organisms. Annu. Rev. Mar. Sci. 12, 291–314. doi: 10.1146/annurev-marine-010419-010641
Ayala, A., Muñoz, M. F., and Argüelles, S. (2014). Lipid peroxidation: production, metabolism, and signaling mechanisms of malondialdehyde and 4-hydroxy-2-nonenal. Oxid. Med. Cell. Longev. 2014:360438. doi: 10.1155/2014/360438
Bachok, Z., Mfilinge, P., and Tsuchiya, M. (2006). Characterization of fatty acid composition in healthy and bleached corals from Okinawa, Japan. Coral Reefs 25, 545–554. doi: 10.1007/s00338-006-0130-9
Baird, A. H., Bhagooli, R., Ralph, P. J., and Takahashi, S. (2008). Coral bleaching: the role of the host. Trends Ecol. Evol. 24, 16–20. doi: 10.1016/j.tree.2008.09.005
Baker, A. C., Glynn, P. W., and Riegl, B. (2008). Climate change and coral reef bleaching : An ecological assessment of long-term impacts, recovery trends and future outlook. Estuar. Coast. Shelf Sci. 80, 435–471. doi: 10.1016/j.ecss.2008.09.003
Beers, R. F., and Sizer, I. W. (1952). A spectrophotometric method for measuring the breakdown of hydrogen peroxide by catalase. J. Biol. Chem. 195, 133–140. doi: 10.1016/s0021-9258(19)50881-x
Bhagooli, R., and Hidaka, M. (2004). Photoinhibition, bleaching susceptibility and mortality in two scleractinian corals, Platygyra ryukyuensis and Stylophora pistillata, in response to thermal and light stresses. Comp. Biochem. Physiol. A 137, 547–555. doi: 10.1016/j.cbpb.2003.11.008
Biel, K. Y., Gates, R. D., and Muscatine, L. (2007). Effects of free amino acids on the photosynthetic carbon metabolism of symbiotic dinoflagellates. Russ. J. Plant Physiol. 54, 171–83. doi: 10.1134/s1021443707020033
Bradford, M. M. (1976). A rapid and sensitive method for the quantitation of microgram quantities of protein utilizing the principle of protein-dye binding. Anal. Biochem. 72, 248–254. doi: 10.1016/0003-2697(76)90527-3
Calder, P. C. (2010). Omega-3 fatty acids and inflammatory processes. Nutrients 2, 355–374. doi: 10.3390/nu2030355
Calder, P. C. (2017). Omega-3 fatty acids and inflammatory processes: from molecules to man. Biochem. Soc. Trans. 45, 1105–1115. doi: 10.1042/BST20160474
Catalá, A. (2010). A synopsis of the process of lipid peroxidation since the discovery of the essential fatty acids. Biochem. Bioph Res. Co. 399, 318–323. doi: 10.1016/j.bbrc.2010.07.087
Cook, C. B., and Davy, S. K. (2001). Are free amino acids responsible for the ‘host factor’ effects on symbiotic zooxanthellae in extracts of host tissue? Hydrobiologia 461, 71–78. doi: 10.1023/A:1012785725378
Cunning, R., and Baker, A. C. (2012). Excess algal symbionts increase the susceptibility of reef corals to bleaching. Nat. Clim. Chang. 3, 259–262. doi: 10.1038/NCLIMATE1711
Cziesielski, M. J., Schmidt-Roach, S., and Aranda, M. (2019). The past, present, and future of coral heat stress studies. Ecol. Evol. 9, 10055–10066. doi: 10.1002/ece3.5576
Dalsgaard, J., John, M. S., Kattner, G., Müller-Navarra, D., and Hagen, W. (2003). Fatty acid trophic markers in the pelagic marine environment. Adv. Mar. Biol. 46, 227–318.
De’ath, G., Fabricius, K. E., Sweatman, H., and Puotinen, M. (2012). The 27 – year decline of coral cover on the Great Barrier Reef and its causes. PNAS 109:1208909109. doi: 10.1073/pnas.1208909109
Dias, M., Ferreira, A., Gouveia, R., Madeira, C., Jogee, N., Cabral, H., et al. (2019). Long-term exposure to increasing temperatures on scleractinian coral fragments reveals oxidative stress. Mar. Environ. Res. 150:104758. doi: 10.1016/j.marenvres.2019.104758
Downs, C. A., Fauth, J. E., Halas, J. C., Dustan, P., Bemiss, J., and Woodley, C. M. (2002). Oxidative stress and seasonal coral bleaching. Free Radic. Biol. Med. 33, 533–543. doi: 10.1016/s0891-5849(02)00907-3
Fitt, W. K., McFarland, F. K., Warner, M. E., and Chilcoat, G. C. (2000). Seasonal patterns of tissue biomass and densities of symbiotic dinoflagellates in reef corals and relation to coral bleaching. Limnol. Oceanogr. 45, 677–685. doi: 10.4319/lo.2000.45.3.0677
Fujise, L., Yamashita, H., Suzuki, G., Sasaki, K., Liao, L. M., and Koike, K. (2014). Moderate thermal stress causes active and immediate expulsion of photosynthetically damaged zooxanthellae (Symbiodinium) from corals. PLoS One 9:e114321. doi: 10.1371/journal.pone.0114321
Grottoli, A. G., Rodrigues, L. J., and Juarez, C. (2004). Lipids and stable carbon isotopes in two species of Hawaiian corals, Porites compressa and Montipora verrucosa, following a bleaching event. Mar. Biol. 145, 621–631. doi: 10.1007/s00227-004-1337-3
Guest, J. R., Baird, A. H., Maynard, J. A., Muttaqin, E., Edwards, A. J., Campbell, S. J., et al. (2012). Contrasting patterns of coral bleaching susceptibility in 2010 suggest an adaptive response to thermal stress. PLoS One 7:e33353.
Habig, W. H., and Jakoby, W. B. (1981). Assays for differentiation of glutathione S-Transferases. Methods Enzymol. 77, 398–405. doi: 10.1016/S0076-6879(81)77053-8
Imbs, A. B., and Dautova, T. N. (2008). Use of lipids for chemotaxonomy of octocorals (Cnidaria: Alcyonaria). Russ. J. Mar. Biol. 34, 174–178.
Imbs, A. B., and Yakovleva, I. M. (2012). Dynamics of lipid and fatty acid composition of shallow-water corals under thermal stress: an experimental approach. Coral Reefs 31, 41–53. doi: 10.1007/s00338-011-0817-4
Imbs, A. B., Latyshev, N. A., Dautova, T. N., and Latypov, Y. Y. (2010a). Distribution of lipids and fatty acids in corals by their taxonomic position and presence of zooxanthellae. Mar. Ecol. Prog. Ser. 409, 65–75. doi: 10.3354/meps08622
Imbs, A. B., Yakovleva, I. M., Dautova, T. N., Bui, L. H., and Jones, P. (2014). Diversity of fatty acid composition of symbiotic dinoflagellates in corals: Evidence for the transfer of host PUFAs to the symbionts. Phytochemistry 101, 76–82. doi: 10.1016/j.phytochem.2014.02.012
Imbs, A. B., Yakovleva, I. M., Latyshev, N. A., and Pham, L. Q. (2010b). Biosynthesis of polyunsaturated fatty acids in zooxanthellae and polyps of corals. Russ. J. Mar. Biol. 36, 452–457. doi: 10.1134/S1063074010060076
Imbs, A. B., Yakovleva, I. M., and Pham, Q. L. (2010c). Distribution of lipids and fatty acids in the zooxanthellae and host of the soft coral Sinularia sp. Fish. Sci. 76, 375–380. doi: 10.1007/s12562-009-0213-y
Innes, J. K., and Calder, P. C. (2018). Omega-6 fatty acids and inflammation. Prostag Leukotr Essential Fatty Acids 132, 41–48. doi: 10.1016/j.plefa.2018.03.004
Johannes, R. E., and Wiebe, W. J. (1970). Method for determination of coral tissue biomass and composition. Limnol. Oceanogr. 15, 822–824. doi: 10.4319/lo.1970.15.5.0822
Kim, T., Lee, J. C., Kang, D. H., Duprey, N. N., Leung, K. S., Archana, A., et al. (2021). Modification of fatty acid profile and biosynthetic pathway in symbiotic corals under eutrophication. Sci. Total Environ. 2021:145336. doi: 10.1016/j.scitotenv.2021.145336
Kumar, N. G., Contaifer, D., Madurantakam, P., Carbone, S., Price, E. T., and Van Tassell (2019). Dietary bioactive fatty acids as modulators of immune function: implications on human health. Nutrients 11:2974. doi: 10.3390/nu11122974
Lesser, M. P. (1997). Oxidative stress causes coral bleaching during exposure to elevated temperatures. Coral Reefs 16, 187–192. doi: 10.1007/s003380050073
Lesser, M. P. (2006). Oxidative stress in marine environments: biochemistry and physiological ecology. Annu. Rev. Physiol. 68, 253–278. doi: 10.1146/annurev.physiol.68.040104.110001
Lesser, M. P. (2011). “Coral bleaching: causes and mechanisms,” in Coral Reefs: An Ecosystem in Transition, eds Z. Dubinsky and N. Stambler (Dordrecht: Springer), 1–552. doi: 10.1007/978-94-007-0114-4_23
Lushchak, V. I. (2011). Environmentally induced oxidative stress in aquatic animals. Aquat. Toxicol. 101, 13–30. doi: 10.1016/j.aquatox.2010.10.006
Mansfield, K. M., and Gilmore, T. D. (2019). Innate immunity and cnidarian-Symbiodiniaceae mutualism. Dev. Comp. Immunol. 90, 199–209. doi: 10.1016/j.dci.2018.09.020
Monroig, Ó, Tocher, D. R., and Navarro, J. C. (2013). Biosynthesis of polyunsaturated fatty acids in marine invertebrates: recent advances in molecular mechanisms. Mar. Drugs 11, 3998–4018. doi: 10.3390/md11103998
Nesa, B., and Hidaka, M. (2009). High zooxanthella density shortens the survival time of coral cell aggregates under thermal stress. J. Exp. Mar. Biol. Ecol. 368, 81–87. doi: 10.1016/j.jembe.2008.10.018
Okuyama, H., Orikasa, Y., and Nishida, T. (2008). Significance of antioxidative functions of eicosapentaenoic and docosahexaenoic acids in marine microorganisms. Appl. Environ. Microbiol. 74, 570–574. doi: 10.1128/AEM.02256-07
Palmer, C. V. (2018). Immunity and the coral crisis. Commun. Biol. 1, 1–7. doi: 10.1038/s42003-018-0097-4
Papina, M., Meziane, T., and Van Woesik, R. (2003). Symbiotic zooxanthellae provide the host-coral Montipora digitata with polyunsaturated fatty acids. Comp. Biochem. Physiol. B Biochem. Mol. Biol. 135, 533–537. doi: 10.1016/S1096-4959(03)00118-0
Papina, M., Meziane, T., and Van Woesik, R. (2007). Acclimation effect on fatty acids of the coral Montipora digitata and its symbiotic algae. Comp. Biochem. Physiol. B Biochem. Mol. Biol. 147, 583–589. doi: 10.1016/j.cbpb.2007.02.011
Parrish, C. C. (2013). Lipids in marine ecosystems. Int. Sch. Res. Notices 2013:604045. doi: 10.5402/2013/604045
Ratnayake, W. N., and Galli, C. (2009). Fat and fatty acid terminology, methods of analysis and fat digestion and metabolism. Ann. Nutr. Metab. 55, 8–43. doi: 10.1159/000228994
Rewitz, K. F., Styrishave, B., Løbner-Olesen, A., and Andersen, O. (2006). Marine invertebrate cytochrome P450: emerging insights from vertebrate and insect analogies. Comp. Biochem. Physiol. C Toxicol. Pharmacol. 143, 363–381. doi: 10.1016/j.cbpc.2006.04.001
Rocker, M. M., Francis, D. S., Fabricius, K. E., Willis, B. L., and Bay, L. K. (2019). Temporal and spatial variation in fatty acid composition in Acropora tenuis corals along water quality gradients on the Great Barrier Reef, Australia. Coral Reefs 38, 215–228. doi: 10.1007/s00338-019-01768-x
Rodrigues, L. J., Grottoli, A. G., and Pease, T. K. (2008). Lipid class composition of bleached and recovering Porites compressa Dana, 1846 and Montipora capitata Dana, 1846 corals from Hawaii. J. Exp. Mar. Bio. Ecol. 358, 136–143. doi: 10.1016/j.jembe.2008.02.004
Rodríguez-Troncoso, A. P., Carpizo-Ituarte, E., and Cupul-Magaña, A. L. (2016). Physiological response to high temperature in the Tropical Eastern Pacific coral Pocillopora verrucosa. Mar. Ecol. 37, 1168–1175. doi: 10.1111/maec.12392
Siebeck, U. E., Marshall, N. J., Klüter, A., and Hoegh-Guldberg, O. (2006). Monitoring coral bleaching using a colour reference card. Coral Reefs 25, 453–460. doi: 10.1007/s00338-006-0123-8
Takahashi, S., and Murata, N. (2008). How do environmental stresses accelerate photoinhibition? Trends Plant Sci. 13, 178–182. doi: 10.1016/j.tplants.2008.01.005
Teece, M. A., Estes, B., Gelsleichter, E., and Lirman, D. (2011). Heterotrophic and autotrophic assimilation of fatty acids by two scleractinian corals, Montastraea faveolata and Porites astreoides. Limnol. Oceanogr. 56, 1285–1296. doi: 10.4319/lo.2011.56.4.1285
Teixeira, T., Diniz, M., Calado, R., and Rosa, R. (2013). Coral physiological adaptations to air exposure: heat shock and oxidative stress responses in Veretillum cynomorium. J. Exp. Mar. Biol. Ecol. 439, 35–41. doi: 10.1016/j.jembe.2012.10.010
Treignier, C., Grover, R., Ferrier-Pages, C., and Tolosa, I. (2008). Effect of light and feeding on the fatty acid and sterol composition of zooxanthellae and host tissue isolated from the scleractinian coral Turbinaria reniformis. Limnol. Oceanogr. 53, 2702–2710. doi: 10.4319/lo.2008.53.6.2702
Weis, V. M. (2008). Cellular mechanisms of Cnidarian bleaching : stress causes the collapse of symbiosis. J. Exp. Biol. 211, 3059–3066. doi: 10.1242/jeb.009597
Xu, L., Yu, K., Li, S., Liu, G., Tao, S., Shi, Q., et al. (2017). Interseasonal and interspecies diversities of Symbiodinium density and effective photochemical efficiency in five dominant reef coral species from Luhuitou fringing reef, northern South China Sea. Coral Reefs 36, 477–487. doi: 10.1007/s00338-016-1532-y
Yakovleva, I. M., Baird, A. H., Yamamoto, H. H., Bhagooli, R., Nonaka, M., and Hidaka, M. (2009). Algal symbionts increase oxidative damage and death in coral larvae at high temperatures. Mar. Ecol. Prog. Ser. 378, 105–112. doi: 10.3354/meps07857
Keywords: stress response, heat-stress, Symbiodiniaceae, fatty acids, oxidative stress, coral bleaching
Citation: Safuan CDM, Samshuri MA, Jaafar SNT, Tan CH and Bachok Z (2021) Physiological Response of Shallow-Water Hard Coral Acropora digitifera to Heat Stress via Fatty Acid Composition. Front. Mar. Sci. 8:715167. doi: 10.3389/fmars.2021.715167
Received: 26 May 2021; Accepted: 02 August 2021;
Published: 03 September 2021.
Edited by:
Davide Seveso, University of Milano-Bicocca, ItalyReviewed by:
Carolina Arruda Freire, Federal University of Paraná, BrazilShiming Peng, East China Sea Fisheries Research Institute, Chinese Academy of Fishery Sciences, China
Copyright © 2021 Safuan, Samshuri, Jaafar, Tan and Bachok. This is an open-access article distributed under the terms of the Creative Commons Attribution License (CC BY). The use, distribution or reproduction in other forums is permitted, provided the original author(s) and the copyright owner(s) are credited and that the original publication in this journal is cited, in accordance with accepted academic practice. No use, distribution or reproduction is permitted which does not comply with these terms.
*Correspondence: Siti Nurtahirah Jaafar, tahirah@umt.edu.my